Key Concepts
The trachea serves as a conduit for ventilation and the clearance of tracheal and bronchial secretions and has an average length of 10-13 cm. The trachea bifurcates at the carina into the right and left main stem bronchi. The right main stem bronchus lies in a more vertical orientation relative to the trachea, whereas the left main stem bronchus lies in a more horizontal orientation.
The periodic exchange of alveolar gas with the fresh gas from the upper airway reoxygenates desaturated blood and eliminates CO2. This exchange is brought about by small cyclic pressure gradients established within the airways. During spontaneous ventilation, these gradients are secondary to variations in intrathoracic pressure; during mechanical ventilation, they are produced by intermittent positive pressure in the upper airway.
The lung volume at the end of a normal exhalation is called functional residual capacity (FRC). At this volume, the inward elastic recoil of the lung approximates the outward elastic recoil of the chest (including resting diaphragmatic tone).
Closing capacity is normally well below FRC, but it rises steadily with age. This increase is probably responsible for the normal age-related decline in arterial O2 tension.
Whereas both forced expiratory volume in 1 sec (FEV1) and forced vital capacity (FVC) are effort dependent, forced midexpiratory flow (FEF25-75%) is more effort independent and may be a more reliable measure of obstruction.
Changes in lung mechanics due to general anesthesia occur shortly after induction. The supine position reduces the FRC by 0.8-1.0 L, and induction of general anesthesia further reduces the FRC by 0.4-0.5 L. FRC reduction is a consequence of alveolar collapse and compression atelectasis due to loss of inspiratory muscle tone, change in chest wall rigidity, and upward shift of the diaphragm.
Local factors are more important than the autonomic system in influencing pulmonary vascular tone. Hypoxia is a powerful stimulus for pulmonary vasoconstriction (the opposite of its systemic effect).
Because alveolar ventilation (
) is normally about 4 L/min and pulmonary capillary perfusion (
) is 5 L/min, the overall
ratio is about 0.8.
Shunting denotes the process whereby desaturated, mixed venous blood from the right heart returns to the left heart without being resaturated with O2 in the lungs. The overall effect of shunting is to decrease (dilute) arterial O2 content; this type of shunt is referred to as right-to-left.
General anesthesia commonly increases venous admixture to 5% to 10%, probably as a result of atelectasis and airway collapse in dependent areas of the lung.
Note that large increases in Paco 2 (>75 mm Hg) readily produce hypoxia (Pao 2 <60 mm Hg) at room air, but not at high inspired O2 concentrations.
The binding of O2 to hemoglobin seems to be the principal rate-limiting factor in the transfer of O2 from alveolar gas to blood.
The greater the shunt, the less likely the possibility that an increase in the fraction of inspired oxygen (Fio 2) will prevent hypoxemia.
A rightward shift in the oxygen-hemoglobin dissociation curve lowers O2 affinity, displaces O2 from hemoglobin, and makes more O2 available to tissues; a leftward shift increases hemoglobin’s affinity for O2, reducing its availability to tissues.
Bicarbonate represents the largest fraction of CO2 in blood.
Central chemoreceptors are thought to lie on the anterolateral surface of the medulla and respond primarily to changes in cerebrospinal fluid (CSF) [H+]. This mechanism is effective in regulating Paco 2, because the blood-brain barrier is permeable to dissolved CO2 but not to bicarbonate ions.
With increasing depth of anesthesia, the slope of the Paco 2/minute ventilation curve decreases, and the apneic threshold increases.
Respiratory Physiology & Anesthesia: Introduction
The importance of pulmonary physiology to anesthetic practice is obvious. The most commonly used anesthetics—the inhalation agents—depend on the lungs for uptake and elimination. The most important side effects of both inhalation and intravenously administered anesthetics are primarily respiratory. Moreover, muscle paralysis, unusual positioning during surgery, and techniques such as one-lung anesthesia and cardiopulmonary bypass profoundly alter normal pulmonary physiology.
Much of modern anesthetic practice is based on a thorough understanding of pulmonary physiology and may be considered applied pulmonary physiology. This chapter reviews the basic pulmonary concepts necessary for understanding and applying anesthetic techniques. Although the pulmonary effects of each of the various anesthetic agents are discussed elsewhere in the book, this chapter also reviews the overall effects of general anesthesia on lung function.
The rib cage contains the two lungs, each surrounded by its own pleura. The apex of the chest is small, allowing only for entry of the trachea, esophagus, and blood vessels, whereas the base is formed by the diaphragm. Contraction of the diaphragm—the principal pulmonary muscle—causes the base of the thoracic cavity to descend 1.5-7 cm and its contents (the lungs) to expand. Diaphragmatic movement normally accounts for 75% of the change in chest volume. Accessory respiratory muscles also increase chest volume (and lung expansion) by their action on the ribs. Each rib (except for the last two) articulates posteriorly with a vertebra and is angulated downward as it attaches anteriorly to the sternum. Upward and outward rib movement expands the chest.
During normal breathing, the diaphragm, and, to a lesser extent, the external intercostal muscles are responsible for inspiration; expiration is generally passive. With increasing effort, the sternocleidomastoid, scalene, and pectoralis muscles can be recruited during inspiration. The sternocleidomastoid muscles assist in elevating the rib cage, whereas the scalene muscles prevent inward displacement of the upper ribs during inspiration. The pectoralis muscles can assist chest expansion when the arms are placed on a fixed support. Expiration is normally passive in the supine position, but becomes active in the upright position and with increased effort. Exhalation may be facilitated by the abdominal muscles (rectus abdominis, external and internal oblique, and transversus) and perhaps the internal intercostal muscles—aiding the downward movement of the ribs.
Although not usually considered respiratory muscles, some pharyngeal muscles are important in maintaining the patency of the airway. Tonic and reflex inspiratory activity in the genioglossus keeps the tongue away from the posterior pharyngeal wall. Tonic activity in the levator palati, tensor palati, palatopharyngeus, and palatoglossus prevents the soft palate from falling back against the posterior pharynx, particularly in the supine position.
The trachea serves as a conduit for ventilation and the clearance of tracheal and bronchial secretions. The trachea begins at the lower border of the cricoid cartilage and extends to the level of the carina and has an average length of 10-13 cm. It is composed of C-shaped cartilaginous rings, which form the anterior and lateral walls of the trachea and are connected posteriorly by the membranous wall of the trachea. The external diameters of the trachea measure approximately 2.3 cm coronally and 1.8 cm sagitally in men, with corresponding values of 2.0 cm and 1.4 cm, respectively, in women. The cricoid cartilage is the narrowest part of the trachea, with an average diameter of 17 mm in men and 13 mm in women.
The trachea bifurcates at the carina into the right and left main stem bronchi. The tracheal lumen narrows slightly as it progresses toward the carina, with the tracheal bifurcation located at the level of the sternal angle. The right main stem bronchus lies in a more vertical orientation relative to the trachea, whereas the left main stem bronchus lies in a more horizontal orientation. The right main stem bronchus continues as the bronchus intermedius after the take-off of the right upper lobe bronchus. The distance from the tracheal carina to the take-off of the right upper lobe bronchus is an average of 2.0 cm in men and approximately 1.5 cm in women. One in every 250 individuals in the general population may have an abnormal take-off of the right upper lobe bronchus emerging from above the tracheal carina on the right side. The left main stem bronchus is longer than the right main stem bronchus and measures an average of 5.0 cm in men and 4.5 cm in women. The left main stem bronchus divides into the left upper lobe bronchus and the left lower lobe bronchus.
Humidification and filtering of inspired air are functions of the upper airway (nose, mouth, and pharynx). The function of the tracheobronchial tree is to conduct gas flow to and from the alveoli. Dichotomous division (each branch dividing into two smaller branches), starting with the trachea and ending in alveolar sacs, is estimated to involve 23 divisions, or generations (Figure 23-1). With each generation, the number of airways is approximately doubled. Each alveolar sac contains, on average, 17 alveoli. An estimated 300 million alveoli provide an enormous membrane (50-100 m2) for gas exchange in the average adult.
Figure 23-1
A: Dichotomous division of the airways. (Reproduced, with permission, from Guyton AC: Textbook of Medical Physiology, 7th ed. W.B. Saunders, 1986.) B: The segmental bronchi. (Reproduced, with permission, from Minnich DJ, Mathisen DJ: Anatomy of the trachea, carina, and bronchi. Thorac Surg Clin 2007 Nov;17(4):571-585.)
With each successive division, the mucosal epithelium and supporting structures of the airways gradually change. The mucosa makes a gradual transition from ciliated columnar to cuboidal and finally to flat alveolar epithelium. Gas exchange can occur only across the flat epithelium, which begins to appear on respiratory bronchioles (generations 17-19). The wall of the airway gradually loses its cartilaginous support (at the bronchioles) and then its smooth muscle. Loss of cartilaginous support causes the patency of smaller airways to become dependent on radial traction by the elastic recoil of the surrounding tissue; as a corollary, airway diameter becomes dependent on total lung volume.
Cilia on the columnar and cuboidal epithelium normally beat in a synchronized fashion, such that the mucus produced by the secretory glands lining the airway (and any associated bacteria or debris) moves up toward the mouth.
Alveolar size is a function of both gravity and lung volume. The average diameter of an alveolus is thought to be 0.05-0.33 mm. In the upright position, the largest alveoli are at the pulmonary apex, whereas the smallest tend to be at the base. With inspiration, discrepancies in alveolar size diminish.
Each alveolus is in close contact with a network of pulmonary capillaries. The walls of each alveolus are asymmetrically arranged (Figure 23-2). On the thin side, where gas exchange occurs, the alveolar epithelium and capillary endothelium are separated only by their respective cellular and basement membranes; on the thick side, where fluid and solute exchange occurs, the pulmonary interstitial space separates alveolar epithelium from capillary endothelium. The pulmonary interstitial space contains mainly elastin, collagen, and perhaps nerve fibers. Gas exchange occurs primarily on the thin side of the alveolocapillary membrane, which is less than 0.4 μm thick. The thick side (1-2 μm) provides structural support for the alveolus.
Figure 23-2
The pulmonary interstitial space, with a capillary passing between the two alveoli. The capillary is incorporated into the thin (gas-exchanging) side of the alveolus on the right. The interstitial space is incorporated into the thick side of the alveolus on the left. (Reproduced, with permission, from Nunn JF: Nunn’s Applied Physiology, 4th ed. Butterworth, 2000.)
The pulmonary epithelium contains at least two cell types. Type I pneumocytes are flat and form tight (1-nm) junctions with one another. These tight junctions are important in preventing the passage of large oncotically active molecules such as albumin into the alveolus. Type II pneumocytes, which are more numerous than type I pneumocytes (but because of their shape occupy less than 10% of the alveolar space), are round cells that contain prominent cytoplasmic inclusions (lamellar bodies). These inclusions contain surfactant, an important substance necessary for normal pulmonary mechanics (see below). Unlike type I cells, type II pneumocytes are capable of cell division and can produce type I pneumocytes if the latter are destroyed. They are also resistant to O2 toxicity.
Other cell types present in the lower airways include pulmonary alveolar macrophages, mast cells, lymphocytes, and amino precursor uptake and decarboxylation (APUD) cells. Neutrophils are also typically present in smokers and patients with acute lung injury.
The lungs are supplied by two circulations, pulmonary and bronchial. The bronchial circulation arises from the left heart and sustains the metabolic needs of the tracheobronchial tree. The bronchial circulation provides a small amount of blood flow (ie, less than 4% of the cardiac output). Branches of the bronchial artery supply the wall of the bronchi and follow the airways as far as the terminal bronchioles. Along their courses, the bronchial vessels anastomose with the pulmonary arterial circulation and continue as far as the alveolar duct. Below that level, lung tissue is supported by a combination of the alveolar gas and pulmonary circulation. Except for the main bronchi within the mediastinum, almost all the blood carried by the bronchial arteries enters the pulmonary circulation.
The pulmonary circulation normally receives the total output of the right heart via the pulmonary artery, which divides into right and left branches to supply each lung. Deoxygenated blood passes through the pulmonary capillaries, where O2 is taken up and CO2 is eliminated. The oxygenated blood is then returned to the left heart by four main pulmonary veins (two from each lung). Although flows through the systemic and pulmonary circulations are equal, the lower pulmonary vascular resistance results in pulmonary vascular pressures that are one-sixth of those in the systemic circulation; as a result, both pulmonary arteries and veins normally have thinner walls than systemic vessels with less smooth muscle.
There are connections between the bronchial and the pulmonary circulations. Direct pulmonary arteriovenous communications, bypassing the pulmonary capillaries, are normally insignificant but may become important in certain pathological states. The importance of the bronchial circulation in contributing to the normal venous admixture is discussed below.
Pulmonary capillaries are incorporated into the walls of alveoli. The average diameter of these capillaries (about 10 μm) is barely enough to allow passage of a single red cell. Because each capillary network supplies more than one alveolus, blood may pass through several alveoli before reaching the pulmonary veins. Because of the relatively low pressure in the pulmonary circulation, the amount of blood flowing through a given capillary network is affected by both gravity and alveolar size. Large alveoli have a smaller capillary cross-sectional area and consequently increased resistance to blood flow. In the upright position, apical capillaries tend to have reduced flows, whereas basal capillaries have higher flows.
The pulmonary capillary endothelium has relatively large junctions (5 nm wide), allowing the passage of large molecules such as albumin. As a result, pulmonary interstitial fluid is relatively rich in albumin. Circulating macrophages and neutrophils are able to pass through the endothelium, as well as the smaller alveolar epithelial junctions, with relative ease. Pulmonary macrophages are commonly seen in the interstitial space and inside alveoli; they serve to prevent bacterial infection and to scavenge foreign particles.
Lymphatic channels in the lung originate in the interstitial spaces of large septa and are close to the bronchial arteries. Bronchial lymphatics return fluids, lost proteins, and various cells that have escaped in the peribronchovascular interstitium into the blood circulation, thus ensuring homeostasis and permitting lung function. Because of the large endothelial junctions, pulmonary lymph has a relatively high protein content, and total pulmonary lymph flow may be as much as 20 mL/hr. Large lymphatic vessels travel upward alongside the airways, forming the tracheobronchial chain of lymph nodes. Lymphatic drainage channels from both lungs communicate along the trachea.
The diaphragm is innervated by the phrenic nerves, which arise from the C3-C5 nerve roots. Unilateral phrenic nerve block or palsy only modestly reduces most indices of pulmonary function (about 25%) in normal subjects. Although bilateral phrenic nerve palsies produce more severe impairment, accessory muscle activity may maintain adequate ventilation in some patients. Intercostal muscles are innervated by their respective thoracic nerve roots. Cervical cord injuries above C5 are incompatible with spontaneous ventilation because both phrenic and intercostal nerves are affected.
The vagus nerves provide sensory innervation to the tracheobronchial tree. Both sympathetic and parasympathetic autonomic innervation of bronchial smooth muscle and secretory glands is present. Vagal activity mediates bronchoconstriction and increases bronchial secretions via muscarinic receptors. Sympathetic activity (T1-T4) mediates bronchodilation and also decreases secretions via β2-receptors. The nerve supply of the larynx is reviewed in Chapter 19.
Both α- and β-adrenergic receptors are present in the pulmonary vasculature, but the sympathetic system normally has little effect on pulmonary vascular tone. α1-Activity causes vasoconstriction; β2-activity mediates vasodilation. Parasympathetic vasodilatory activity seems to be mediated via the release of nitric oxide.
The periodic exchange of alveolar gas with the fresh gas from the upper airway reoxygenates desaturated blood and eliminates CO2. This exchange is brought about by small cyclic pressure gradients established within the airways. During spontaneous ventilation, these gradients are secondary to variations in intrathoracic pressure; during mechanical ventilation, they are produced by intermittent positive pressure in the upper airway.
Normal pressure variations during spontaneous breathing are shown in Figure 23-3. The pressure within alveoli is always greater than the surrounding (intrathoracic) pressure unless the alveoli are collapsed. Alveolar pressure is normally atmospheric (zero for reference) at end-inspiration and end-expiration. By convention in pulmonary physiology, pleural pressure is used as a measure of intrathoracic pressure. Although it may not be entirely correct to refer to the pressure in a potential space, the concept allows the calculation of transpulmonary pressure. Transpulmonary pressure, or Ptranspulmonary, is then defined as follows:
Ptranspulmonary = Palveolar − Pintrapleural
At end-expiration, intrapleural pressure normally averages about −5 cm H2O, and because alveolar pressure is 0 (no flow), transpulmonary pressure is +5 cm H2O.
Diaphragmatic and intercostal muscle activation during inspiration expands the chest and decreases intrapleural pressure from −5 cm H2O to −8 or −9 cm H2O. As a result, alveolar pressure also decreases (between −3 and −4 cm H2O), and an alveolar-upper airway gradient is established; gas flows from the upper airway into alveoli. At end-inspiration (when gas inflow has ceased), alveolar pressure returns to zero, but intrapleural pressure remains decreased; the new transpulmonary pressure (5 cm H2O) sustains lung expansion.
During expiration, diaphragmatic relaxation returns intrapleural pressure to −5 cm H2O. Now the transpulmonary pressure does not support the new lung volume, and the elastic recoil of the lung causes a reversal of the previous alveolar-upper airway gradient; gas flows out of alveoli, and original lung volume is restored.
Most forms of mechanical ventilation intermittently apply positive airway pressure at the upper airway. During inspiration, gas flows into alveoli until alveolar pressure reaches that in the upper airway. During the expiratory phase of the ventilator, the positive airway pressure is removed or decreased; the gradient reverses, allowing gas flow out of alveoli.
The movement of the lungs is passive and determined by the impedance of the respiratory system, which can be divided into the elastic resistance of tissues and the gas-liquid interface and the nonelastic resistance to gas flow. Elastic resistance governs lung volume and the associated pressures under static conditions (no gas flow). Resistance to gas flow relates to frictional resistance to airflow and tissue deformation. The work necessary to overcome elastic resistance is stored as potential energy, but the work necessary to overcome nonelastic resistance is lost as heat.
Both the lungs and the chest have elastic properties. The chest has a tendency to expand outward, whereas the lungs have a tendency to collapse. When the chest is exposed to atmospheric pressure (open pneumothorax), it usually expands about 1 L in adults. In contrast, when the lung is exposed to atmospheric pressure, it collapses completely and all the gas within it is expelled. The recoil properties of the chest are due to structural components that resist deformation and chest wall muscle tone. The elastic recoil of the lungs is due to their high content of elastin fibers, and, even more important, the surface tension forces acting at the air-fluid interface in alveoli.
The gas-fluid interface lining the alveoli causes them to behave as bubbles. Surface tension forces tend to reduce the area of the interface and favor alveolar collapse. Laplace’s law can be used to quantify these forces:
The pressure derived from the equation is that within the alveolus. Alveolar collapse is therefore directly proportional to surface tension. Fortunately, in contrast to a bubble, pulmonary surfactant decreases alveolar surface tension. Moreover, the ability of the surfactant to lower surface tension is directly proportional to its concentration within the alveolus, resulting in lower intraalveolar pressure in smaller alveoli. As alveoli become smaller, the surfactant within becomes more concentrated, and surface tension is more effectively reduced. Conversely, when alveoli are overdistended, surfactant becomes less concentrated, and surface tension increases. The net effect is to stabilize alveoli; small alveoli are prevented from getting smaller, whereas large alveoli are prevented from getting larger.
Elastic recoil is usually measured in terms of compliance (C), which is defined as the change in volume divided by the change in distending pressure. Compliance measurements can be obtained for either the chest, the lung, or both together (Figure 23-4). In the supine position, chest wall compliance (Cw) is reduced because of the weight of the abdominal contents against the diaphragm. Measurements are usually obtained under static conditions, (ie, at equilibrium). (Dynamic lung compliance [Cdyn,L], which is measured during rhythmic breathing, is also dependent on airway resistance.) Lung compliance (CL) is defined as
CL is normally 150-200 mL/cm H2O. A variety of factors, including lung volume, pulmonary blood volume, extravascular lung water, and pathological processes (eg, inflammation and fibrosis) affect CL
where transthoracic pressure equals atmospheric pressure minus intrapleural pressure.
Normal chest wall compliance is 200 mL/cm H2O. Total compliance (lung and chest wall together) is 100 mL/cm H2O and is expressed by the following equation:
Lung volumes are important parameters in respiratory physiology and clinical practice (Table 23-1 and Figure 23-5). The sum of all of the named lung volumes equals the maximum to which the lung can be inflated. Lung capacities are clinically useful measurements that represent a combination of two or more volumes.
Measurement | Definition | Average Adult Values (mL) |
---|---|---|
Tidal volume (VT) | Each normal breath | 500 |
Inspiratory reserve volume (IRV) | Maximal additional volume that can be inspired above VT | 3000 |
Expiratory reserve volume (ERV) | Maximal volume that can be expired below VT | 1100 |
Residual volume (RV) | Volume remaining after maximal exhalation | 1200 |
Total lung capacity (TLC) | RV + ERV + VT + IRV | 5800 |
Functional residual capacity (FRC) | RV + ERV | 2300 |
The lung volume at the end of a normal exhalation is called functional residual capacity (FRC). At this volume, the inward elastic recoil of the lung approximates the outward elastic recoil of the chest (including resting diaphragmatic tone). Thus, the elastic properties of both chest and lung define the point from which normal breathing takes place. Functional residual capacity can be measured by nitrogen washout or helium washin technique or by body plethysmography. Factors known to alter the FRC include the following:
- Body habitus: FRC is directly proportional to height. Obesity, however, can markedly decrease FRC (primarily as a result of reduced chest compliance).
- Sex: FRC is reduced by about 10% in females compared with males.
- Posture: FRC decreases as a patient is moved from an upright to a supine or prone position. This is the result of reduced chest compliance as the abdominal contents push up against the diaphragm. The greatest change occurs between 0° and 60° of inclination. No further decrease is observed with a head-down position of up to 30°.
- Lung disease: Decreased compliance of the lung, chest, or both is characteristic of restrictive pulmonary disorders all of which are associated with a low FRC.
- Diaphragmatic tone: This normally contributes to FRC.
As described above (see the section on Functional Respiratory Anatomy), small airways lacking cartilaginous support depend on radial traction caused by the elastic recoil of surrounding tissue to keep them open; patency of these airways, particularly in basal areas of the lung, is highly dependent on lung volume. The volume at which these airways begin to close in dependent areas of the lung is called the closing capacity. At lower lung volumes, alveoli in dependent areas continue to be perfused but are no longer ventilated; intrapulmonary shunting of deoxygenated blood promotes hypoxemia (see below).
Closing capacity is usually measured using a tracer gas (xenon-133), which is inhaled near residual volume and then exhaled from total lung capacity.
Closing capacity is normally well below FRC (Figure 23-6), but rises steadily with age (Figure 23-7). This increase is probably responsible for the normal age-related decline in arterial O2 tension. At an average age of 44 years, closing capacity equals FRC in the supine position; by age 66, closing capacity equals or exceeds FRC in the upright position in most individuals. Unlike FRC, closing capacity is unaffected by posture.
Vital capacity (VC) is the maximum volume of gas that can be exhaled following maximal inspiration. In addition to body habitus, VC is also dependent on respiratory muscle strength and chest-lung compliance. Normal VC is about 60-70 mL/kg.
Gas flow in the lung is a mixture of laminar and turbulent flow. Laminar flow can be thought of as consisting of concentric cylinders of gas flowing at different velocities; velocity is highest in the center and decreases toward the periphery. During laminar flow,
where Raw is airway resistance.
Turbulent flow is characterized by random movement of the gas molecules down the air passages. Mathematical description of turbulent flow is considerably more complex:
Resistance is not constant but increases in proportion to gas flow. Moreover, resistance is directly proportional to gas density and inversely proportional to the fifth power of the radius. As a result, turbulent gas flow is extremely sensitive to airway caliber.
Turbulence generally occurs at high gas flows, at sharp angles or branching points, and in response to abrupt changes in airway diameter. Whether turbulent or laminar flow occurs can be predicted by the Reynolds number, which results from the following equation:
A low Reynolds number (<1000) is associated with laminar flow, whereas a high value (>1500) produces turbulent flow. Laminar flow normally occurs only distal to small bronchioles (<1 mm). Flow in larger airways is probably turbulent. Of the gases used clinically, only helium has a significantly lower density-to-viscosity ratio, making it useful clinically during severe turbulent flow (as caused by upper airway obstruction). A helium-O2 mixture not only is less likely to cause turbulent flow but also reduces airway resistance when turbulent flow is present (Table 23-2).
Normal total airway resistance is about 0.5-2 cm H2O/L/sec, with the largest contribution coming from medium-sized bronchi (before the seventh generation). Resistance in large bronchi is low because of their large diameters, whereas resistance in small bronchi is low because of their large total cross-sectional area. The most important causes of increased airway resistance include bronchospasm, secretions, and mucosal edema as well as volume-related and flow-related airway collapse.
At low lung volumes, loss of radial traction increases the contribution of small airways to total resistance; airway resistance becomes inversely proportional to lung volume (Figure 23-8). Increasing lung volume up to normal with positive end-expiratory pressure (PEEP) can reduce airway resistance.
During forced exhalation, reversal of the normal transmural airway pressure can cause collapse of these airways (dynamic airway compression). Two contributing factors are responsible: generation of a positive pleural pressure and a large pressure drop across intrathoracic airways as a result of increased airway resistance. The latter is in turn due to high (turbulent) gas flow and the reduced lung volume. The terminal portion of the flow/volume curve is therefore considered to be effort independent (Figure 23-9).
Figure 23-9
Gas flow (A) during forced exhalation from total lung capacity with varying effort and (B) with maximal effort from different lung volumes. Note that regardless of initial lung volume or effort, terminal expiratory flows are effort independent. (Reproduced, with permission, from Nunn JF: Nunn’s Applied Physiology, 4th ed. Butterworth, 2000.)
The point along the airways where dynamic compression occurs is called the equal pressure point. It is normally beyond the eleventh to thirteenth generation of bronchioles where cartilaginous support is absent (see above). The equal pressure point moves toward smaller airways as lung volume decreases. Emphysema or asthma predisposes patients to dynamic airway compression. Emphysema destroys the elastic tissues that normally support smaller airways. In patients with asthma, bronchoconstriction and mucosal edema intensify airway collapse and promote reversal of transmural pressure gradients across airways. Patients may terminate exhalation prematurely or purse their lips to increase expiratory resistance at the mouth. Premature termination of exhalation may increase FRC above normal, resulting in air trapping and auto-PEEP.
Measuring vital capacity as an exhalation that is as forceful and rapid as possible (Figure 23-10) provides important information about airway resistance. The ratio of the forced expiratory volume in the first second of exhalation (FEV1) to the total forced vital capacity (FVC) is proportional to the degree of airway obstruction. Normally, FEV1/FVC is ≥80%. Whereas both FEV1 and FVC are effort dependent, forced midexpiratory flow (FEF25-75%) is more effort independent and may be a more reliable measurement of obstruction.
This component of nonelastic resistance is generally underestimated and often overlooked, but may account for up to half of total airway resistance. It seems to be primarily due to viscoelastic (frictional) resistance of tissues to gas flow.
Because expiration is normally entirely passive, both the inspiratory and the expiratory work of breathing is performed by the inspiratory muscles (primarily the diaphragm). Three factors must be overcome during ventilation: the elastic recoil of the chest and lung, frictional resistance to gas flow in the airways, and tissue frictional resistance.
Respiratory work can be expressed as the product of volume and pressure (Figure 23-11). During inhalation, both inspiratory airway resistance and pulmonary elastic recoil must be overcome; nearly 50% of the energy expended is stored pulmonary elastic recoil. During exhalation, the stored potential energy is released and overcomes expiratory airway resistance. Increases in either inspiratory or expiratory resistance are compensated by increased inspiratory muscle effort. When expiratory resistance increases, the normal compensatory response is to increase lung volume such that Vt breathing occurs at an abnormally high FRC. The greater elastic recoil energy stored at a higher lung volume overcomes the added expiratory resistance. Excessive amounts of expiratory resistance also activate expiratory muscles (see above).
Respiratory muscles normally account for only 2% to 3% of O2 consumption but operate at about 10% efficiency. Ninety percent of the work is dissipated as heat (due to elastic and airflow resistance). In pathological conditions that increase the load on the diaphragm, muscle efficiency usually progressively decreases, and contraction may become uncoordinated with increasing ventilatory effort; moreover, a point may be reached whereby any increase in O2 uptake (because of augmented ventilation) is consumed by the respiratory muscles themselves.
The work required to overcome elastic resistance increases as VT increases, whereas the work required to overcome airflow resistance increases as respiratory rate (and, necessarily, expiratory flow) increases. Faced with either condition, patients minimize the work of breathing by altering the respiratory rate and VT (Figure 23-12). Patients with reduced compliance tend to have rapid, shallow breaths, whereas those with increased airflow resistance have a slow, deep breathing pattern.
The effects of anesthesia on breathing are complex and relate to changes both in position and anesthetic agent.
Changes in lung mechanics due to general anesthesia occur shortly after induction. The supine position reduces the FRC by 0.8-1.0 L, and induction of general anesthesia further reduces the FRC by 0.4-0.5 L. FRC reduction is a consequence of alveolar collapse and compression atelectasis due to loss of inspiratory muscle tone, change in chest wall rigidity, and upward shift of the diaphragm. The mechanisms may be more complex; for example, only the dependent (dorsal) part of the diaphragm in the supine position moves cephalad. Other factors are likely due to a change in intrathoracic volume secondary to increased blood volume in the lung and changes in chest wall shape (Figure 23-13). The higher position of the dorsal diaphragm and changes in the thoracic cavity itself decrease lung volumes. This decrease in FRC is not related to anesthetic depth and may persist for several hours or days after anesthesia. Steep head-down (Trendelenburg) position (>30°) may reduce FRC even further as intrathoracic blood volume increases. In contrast, induction of anesthesia in the sitting position seems to have little effect on FRC. Muscle paralysis does not seem to change FRC significantly when the patient is already anesthetized.
Figure 23-13
With induction of anesthesia in the supine position, the abdominal contents exert cephalad pressure on the diaphragm. At end-expiration, the dorsal portion of the diaphragm is more cephalad and the ventral portion is more caudal than when awake, the thoracic spine is more lordotic, and the rib cage moves inward, all secondary to loss of motor tone.
The effects of anesthesia on closing capacity are more variable. Both FRC and closing capacity, however, are generally reduced to the same extent under anesthesia. Thus, the risk of increased intrapulmonary shunting under anesthesia is similar to that in the conscious state; it is greatest in the elderly, in obese patients, and in those with underlying pulmonary disease.
The reduction in FRC associated with general anesthesia would be expected to increase airway resistance. Increases in airway resistance are not usually observed, however, because of the bronchodilating properties of the volatile inhalation anesthetics. Increased airway resistance is more commonly due to pathological factors (posterior displacement of the tongue; laryngospasm; bronchoconstriction; or secretions, blood, or tumor in the airway) or equipment problems (small tracheal tubes or connectors, malfunction of valves, or obstruction of the breathing circuit).
Increases in the work of breathing under anesthesia are most often secondary to reduced lung and chest wall compliance, and, less commonly, increases in airway resistance (see above). The problems of increased work of breathing are usually circumvented by controlled mechanical ventilation.
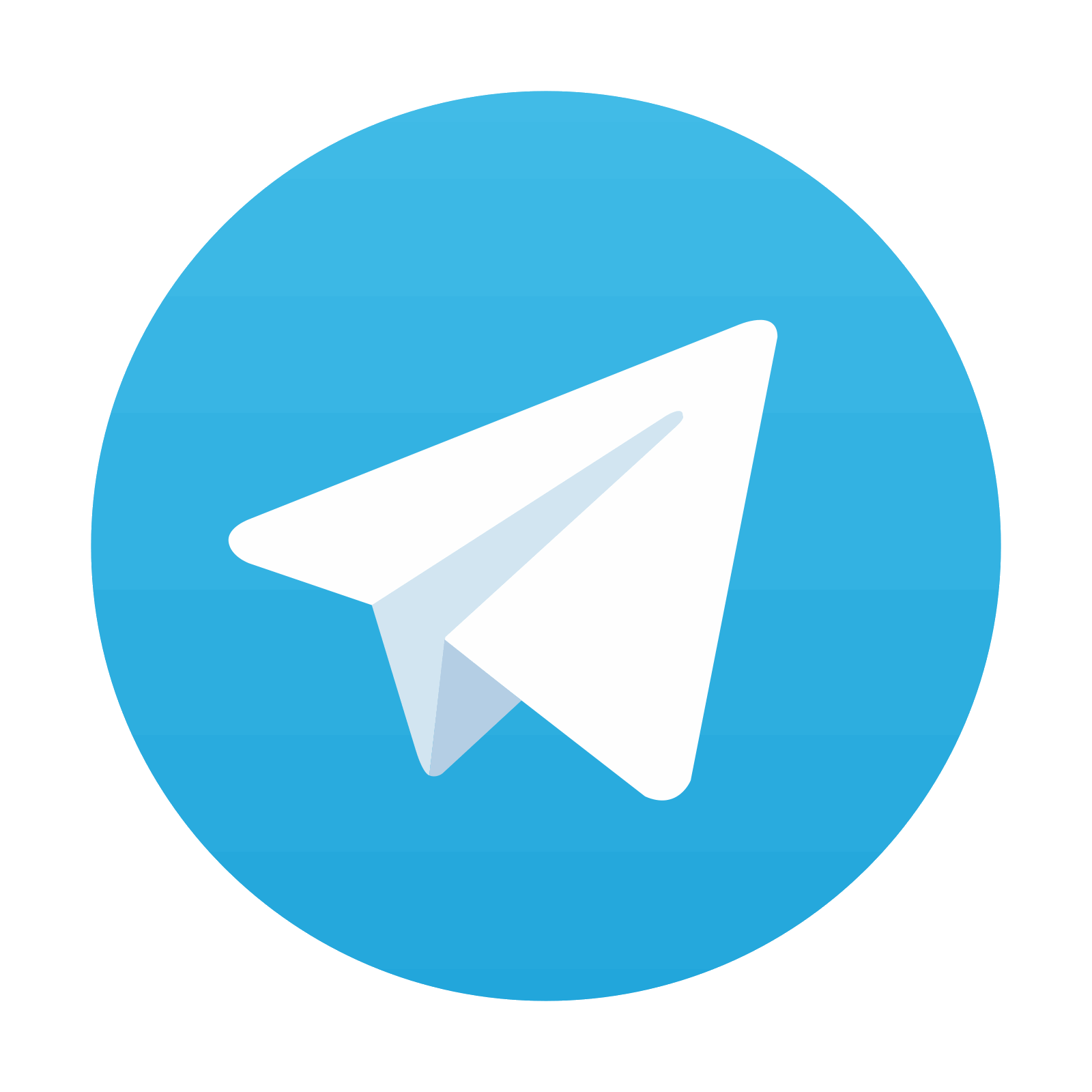
Stay updated, free articles. Join our Telegram channel
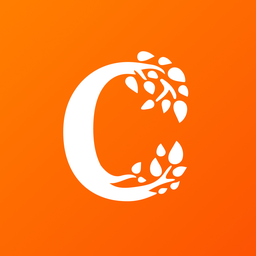
Full access? Get Clinical Tree
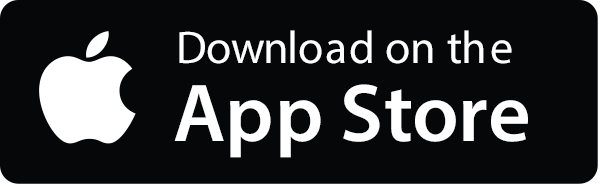
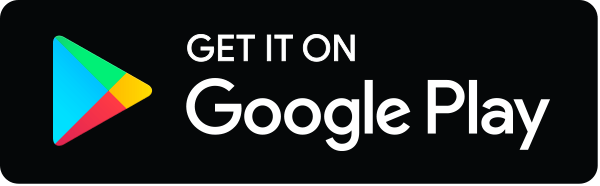