Key Concepts
Sodium (Na) channels are membrane-bound proteins that are composed of one large α subunit, through which Na ions pass, and one or two smaller β subunits. Voltage-gated Na channels exist in (at least) three states—resting (nonconducting), open (conducting), and inactivated (nonconducting). Local anesthetics bind a specific region of the α subunit and inhibit voltage-gated Na channels, preventing channel activation and the Na influx associated with membrane depolarization.
Sensitivity of nerve fibers to inhibition by local anesthetics is determined by axonal diameter, myelination, and other anatomic and physiological factors.
Potency correlates with octanol solubility, which in turn reflects the ability of the local anesthetic molecule to permeate lipid membranes. Potency is increased by adding large alkyl groups to a parent molecule. There is no measurement of local anesthetic potency that is analogous to the minimum alveolar concentration of inhalation anesthetics.
Onset of action depends on many factors, including lipid solubility and the relative concentration of the nonionized lipid-soluble form (B) and the ionized water-soluble form (BH+), expressed by the pKa. The pKa is the pH at which the fraction of ionized and nonionized drug is equal. Less potent, less lipid-soluble agents generally have a faster onset than more potent, more lipid-soluble agents.
Duration of action correlates with potency and lipid solubility. Highly lipid-soluble local anesthetics have a longer duration of action, presumably because they more slowly diffuse from a lipid-rich environment to the aqueous bloodstream.
In regional anesthesia local anesthetics are typically injected or applied very close to their intended site of action; thus their pharmacokinetic profiles are much more important determinants of elimination and toxicity than of their desired clinical effect.
The rate of systemic absorption is related to the vascularity of the site of injection: intravenous (or intraarterial) > tracheal > intercostal > paracervical > epidural > brachial plexus > sciatic > subcutaneous.
Ester local anesthetics are predominantly metabolized by pseudocholinesterase. Amide local anesthetics are metabolized (N-dealkylation and hydroxylation) by microsomal P-450 enzymes in the liver.
The central nervous system is vulnerable to local anesthetic toxicity and is the site of premonitory signs of rising blood concentrations in awake patients.
Major cardiovascular toxicity usually requires about three times the local anesthetic concentration in blood as that required to produce seizures.
Unintentional intravascular injection of bupivacaine during regional anesthesia may produce severe cardiovascular toxicity, including left ventricular depression, atrioventricular heart block, and life-threatening arrhythmias such as ventricular tachycardia and fibrillation.
True hypersensitivity reactions to local anesthetic agents—as distinct from systemic toxicity caused by excessive plasma concentration—are uncommon. Esters appear more likely to induce a true allergic reaction (due to IgG or IgE antibodies) especially if they are derivatives (eg, procaine or benzocaine) of p-aminobenzoic acid, a known allergen.
Local Anesthetics: Introduction
Local and regional anesthesia and analgesia techniques depend on a group of drugs—local anesthetics—that transiently inhibit sensory, motor, or autonomic nerve function, or a combination of these functions, when the drugs are injected or applied near neural tissue. This chapter presents the mechanism of action, structure-activity relationships, and clinical pharmacology of local anesthetic drugs. The more commonly used regional anesthetic techniques are presented in Section IV (see Chapters 45 and 46).
Mechanisms of Local Anesthetic Action
Neurons (and all other living cells) maintain a resting membrane potential of −60 to −70 mV by active transport and passive diffusion of ions. The electrogenic, energy-consuming sodium-potassium pump (Na+-K+-ATPase) couples the transport of three sodium (Na) ions out of the cell for every two potassium (K) ions it moves into the cell. This creates an ionic disequilibrium (concentration gradient) that favors the movement of K ions from an intracellular to an extracellular location, and the movement of Na ions in the opposite direction. The cell membrane is normally much more “leaky” to K ions than to Na ions, so a relative excess of negatively charged ions (anions) accumulates intracellularly. This accounts for the negative resting potential difference (-70 mV polarization).
Unlike most other types of tissue, excitable cells (eg, neurons or cardiac myocytes) have the capability of generating action potentials. Membrane-bound, voltage-gated Na channels in peripheral nerve axons can produce and transmit membrane depolarizations following chemical, mechanical, or electrical stimuli. When a stimulus is sufficient to depolarize a patch of membrane, the signal can be transmitted as a wave of depolarization along the nerve membrane (an impulse). Activation of voltage-gated Na channels causes a very brief (roughly 1 msec) change in the conformation of the channel, allowing an influx of Na ions and generating an action potential (Figure 16-1). The increase in Na permeability causes temporary depolarization of the membrane potential to +35 mV. The Na current is brief and is terminated by inactivation of voltage-gated Na channels, which do not conduct Na ions. Subsequently the membrane returns to its resting potential. Baseline concentration gradients are maintained by the sodium-potassium pump, and only a minuscule number of Na ions pass into the cell during an action potential.
Figure 16-1
Compound Aα, Aδ, and C fiber action potentials recorded after supramaximal stimulation of a rat sciatic nerve. Note the differing time scale of the recordings. In peripheral nerves, Aδ and C fibers have much slower conduction velocities, and their compound action potentials are longer and of less amplitude when compared with those from Aα fibers. (Reproduced, with permission, from Butterworth JF 4th, Strichartz GR: The alpha 2-adrenergic agonists clonidine and guanfacine produce tonic and phasic block of conduction in rat sciatic nerve fibers. Anesth Analg 1993;76:295.)
Na channels are membrane-bound proteins that are composed of one large α subunit, through which Na ions pass, and one or two smaller β subunits. Voltage-gated Na channels exist in (at least) three states—resting (nonconducting), open (conducting), and inactivated (nonconducting) (Figure 16-2). Local anesthetics bind a specific region of the α subunit and inhibit voltage-gated Na channels, preventing channel activation and inhibiting the Na influx associated with membrane depolarization. Local anesthetic binding to Na channels does not alter the resting membrane potential. With increasing local anesthetic concentrations, an increasing fraction of the Na channels in the membrane bind a local anesthetic molecule and cannot conduct Na ions. As a consequence, impulse conduction slows, the rate of rise and the magnitude of the action potential decrease, and the threshold for excitation and impulse conduction increases progressively. At high enough local anesthetic concentrations and with a sufficient fraction of local anesthetic-bound Na channels, an action potential can no longer be generated and impulse propagation is abolished. Local anesthetics have a greater affinity for the channel in the open or inactivated state than in the resting state. Local anesthetic binding to open or inactivated channels, or both, is facilitated by depolarization. The fraction of Na channels that have bound a local anesthetic increases with frequent depolarization (eg, during trains of impulses). This phenomenon is termed use-dependent block. Put another way, local anesthetic inhibition is both voltage and frequency dependent, and is greater when nerve fibers are firing rapidly than with infrequent depolarizations.
Figure 16-2
Voltage-gated sodium (Na) channels exist in (at least) three states—resting, activated (open), and inactivated. Note that local anesthetics bind and inhibit the voltage-gated Na channel from a site that is not directly accessible from outside the cell, interfering with the large transient Na influx associated with membrane depolarization.
Local anesthetics may also bind and inhibit calcium (Ca), K, transient receptor potential vanilloid 1 (TRPV1), and many other channels and receptors. Conversely, other classes of drugs, most notably tricyclic antidepressants (amitriptyline), meperidine, volatile anesthetics, Ca channel blockers, and ketamine, also may inhibit Na channels. Tetrodotoxin is a poison that specifically binds Na channels but at a site on the exterior of the plasma membrane. Human studies are under way with similar toxins to determine whether they might provide effective, prolonged analgesia after local infiltration.
Sensitivity of nerve fibers to inhibition by local anesthetics is determined by axonal diameter, myelination, and other anatomic and physiological factors. Table 16-1 lists the most commonly used classification for nerve fibers. In comparing nerve fibers of the same type, small diameter increases sensitivity to local anesthetics. Thus, larger, faster Aα fibers are less sensitive to local anesthetics than smaller, slower-conducting Aδ fibers, and larger unmyelinated fibers are less sensitive than smaller unmyelinated fibers. On the other hand, small unmyelinated C fibers are relatively resistant to inhibition by local anesthetics as compared with larger myelinated fibers. In spinal nerves local anesthetic inhibition (and conduction failure) generally follows the sequence autonomic > sensory > motor, but at steady state if sensory anesthesia is present all fibers are inhibited.
Fiber Type | Modality Served | Diameter (mm) | Conduction (m/s) | Myelinated? |
---|---|---|---|---|
Aα | Motor efferent | 12-20 | 70-120 | Yes |
Aα | Proprioception | 12-30 | 70-120 | Yes |
Aβ | Touch, pressure | 5-12 | 30-70 | Yes |
Aγ | Motor efferent (muscle spindle) | 3-6 | 15-30 | Yes |
Aδ | Pain | |||
Temperature | ||||
Touch | 2-5 | 12-30 | Yes | |
B | Preganglionic autonomic fibers | <3 | 3-14 | Some |
C Dorsal root | Pain | |||
Temperature | 0.4-1.2 | 0.5-2 | No | |
C Sympathetic | Postganglionic sympathetic fibers | 0.3-1.3 | 0.7-2.3 | No |
Structure-Activity Relationships
Local anesthetics consist of a lipophilic group (usually an aromatic benzene ring) separated from a hydrophilic group (usually a tertiary amine) by an intermediate chain that includes an ester or amide linkage. Articaine, the most popular local anesthetic for dentistry in several European countries, is an amide but it contains a thiophene ring rather than a benzene ring. Local anesthetics are weak bases that usually carry a positive charge at the tertiary amine group at physiological pH. The nature of the intermediate chain is the basis of the classification of local anesthetics as either esters or amides (Table 16-2). Physicochemical properties of local anesthetics depend on the substitutions in the aromatic ring, the type of linkage in the intermediate chain, and the alkyl groups attached to the amine nitrogen.
Generic (Proprietary) | Structure | Relative Lipid Solubility of Unchanged Local Anesthetic | pKa | Protein Binding (%) |
---|---|---|---|---|
Amides | ||||
Bupivacaine (Marcaine, Sensorcaine) | ![]() | 8 | 8.2 | 96 |
Etidocaine (Duranest) | ![]() | 16 | 8.1 | 94 |
Lidocaine (Xylocaine) | ![]() | 1 | 8.2 | 64 |
Mepivacaine (Carbocaine) | ![]() | 0.3 | 7.9 | 78 |
Prilocaine (Citanest) | ![]() | 0.4 | 8.0 | 53 |
Ropivacaine (Naropin) | ![]() | 2.5 | 8.2 | 94 |
Esters | ||||
Chloroprocaine (Nesacaine) | ![]() | 2.3 | 9.1 | NA1 |
Cocaine | ![]() | NA | 8.7 | 91 |
Procaine (Novocaine) | ![]() | 0.3 | 9.1 | NA |
Tetracaine (Pontocaine) | ![]() | 12 | 8.6 | 76 |
Potency correlates with octanol solubility, which in turn reflects the ability of the local anesthetic molecule to permeate lipid membranes. Potency is increased by adding large alkyl groups to a parent molecule (compare tetracaine to procaine or bupivacaine to mepivacaine). There is no measurement of local anesthetic potency that is analogous to the minimum alveolar concentration (MAC) of inhalation anesthetics. The minimum concentration of local anesthetic that will block nerve impulse conduction is affected by several factors, including fiber size, type, and myelination; pH (acidic pH antagonizes block); frequency of nerve stimulation; and electrolyte concentrations (hypokalemia and hypercalcemia antagonize blockade).
Onset of local anesthetic action depends on many factors, including lipid solubility and the relative concentration of the nonionized lipid-soluble form (B) and the ionized water-soluble form (BH+), expressed by the pKa. The pK
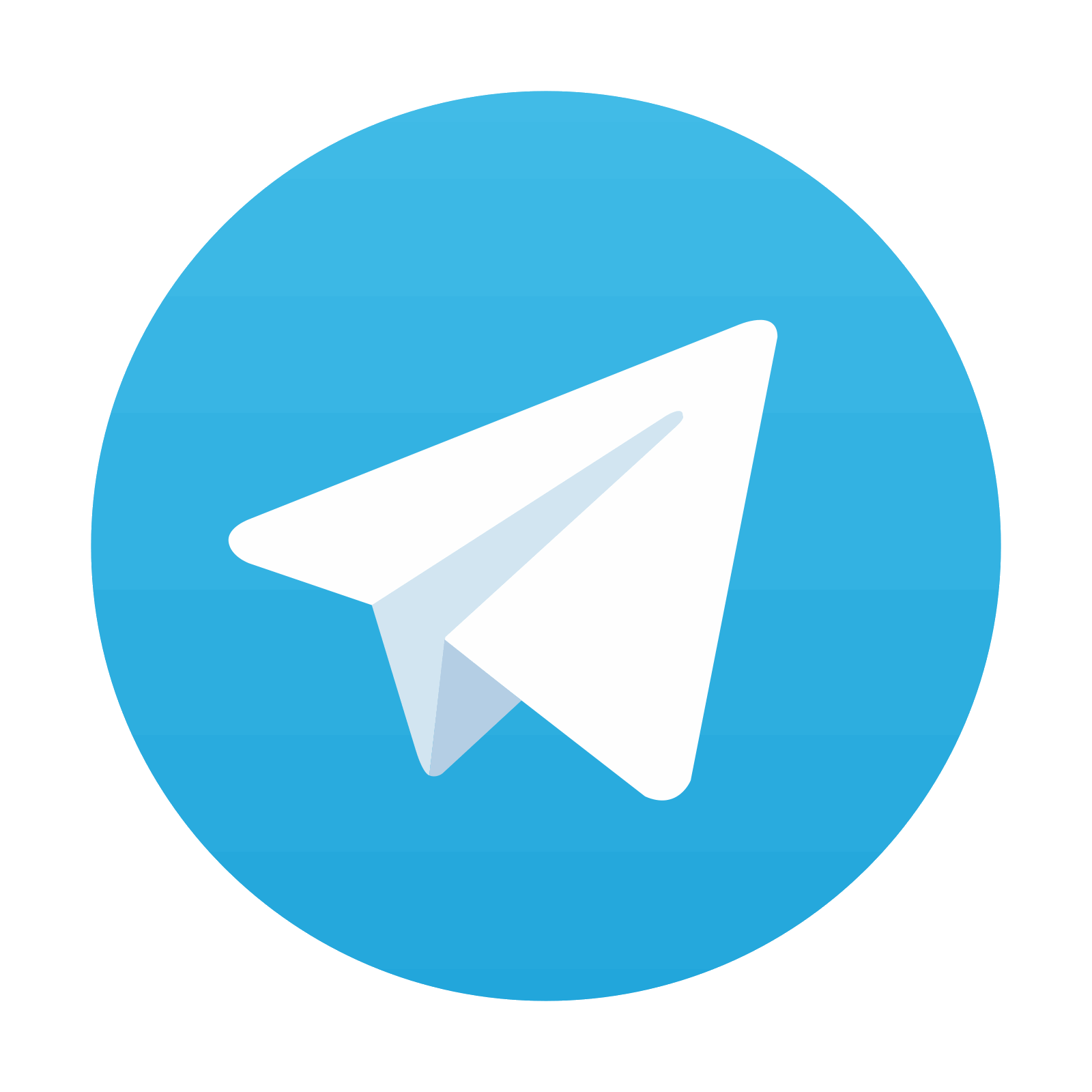
Stay updated, free articles. Join our Telegram channel
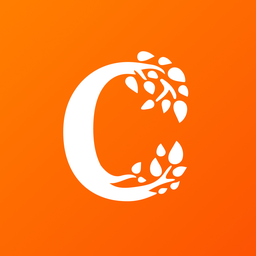
Full access? Get Clinical Tree
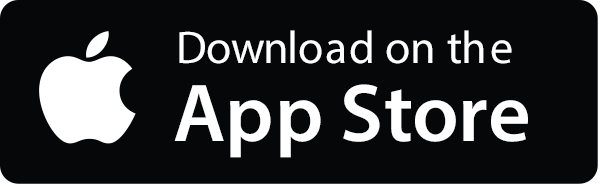
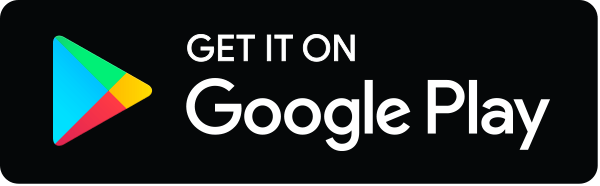
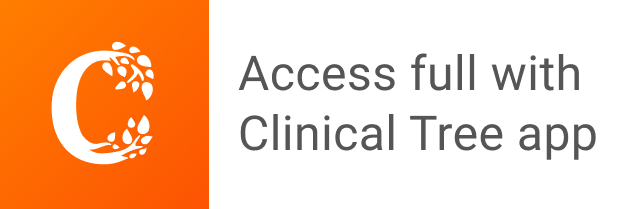