Key Concepts
In contrast to action potentials in axons, the spike in cardiac action potentials is followed by a plateau phase that lasts 0.2-0.3 sec. Whereas the action potential for skeletal muscle and nerves is due to the abrupt opening of voltage-gated sodium channels in the cell membrane, in cardiac muscle it is initiated by voltage-gated sodium channels (the spike) and maintained by voltage-gated calcium channels (the plateau).
Potent inhalational agents depress sinoatrial (SA) node automaticity. These agents seem to have only modest direct effects on the atrioventricular (AV) node, prolonging conduction time and increasing refractoriness. This combination of effects likely explains the frequent occurrence of junctional tachycardia when an anticholinergic agent is administered for sinus bradycardia during inhalation anesthesia; junctional pacemakers are accelerated more than those in the SA node.
Studies suggest that volatile anesthetics depress cardiac contractility by decreasing the entry of Ca2+ into cells during depolarization (affecting T- and L-type calcium channels), altering the kinetics of its release and uptake into the sarcoplasmic reticulum, and decreasing the sensitivity of contractile proteins to calcium.
Because the normal cardiac index (CI) has a wide range, it is a relatively insensitive measurement of ventricular performance. Abnormalities in CI therefore usually reflect gross ventricular impairment.
In the absence of hypoxia or severe anemia, measurement of mixed venous oxygen tension (or saturation) is an excellent estimate of the adequacy of cardiac output.
Patients with reduced ventricular compliance are most affected by loss of a normally timed atrial systole.
Cardiac output in patients with marked right or left ventricular impairment is very sensitive to acute increases in afterload.
The ventricular ejection fraction, the fraction of the end-diastolic ventricular volume ejected, is the most commonly used clinical measurement of systolic function.
Left ventricular diastolic function can be assessed clinically by Doppler echocardiography in a transthoracic or transesophageal examination.
Because the endocardium is subjected to the greatest intramural pressures during systole, it tends to be most vulnerable to ischemia during decreases in coronary perfusion pressure.
The failing heart becomes increasingly dependent on circulating catecholamines. Abrupt withdrawal in sympathetic outflow or decreases in circulating catecholamine levels, such as can occur following induction of anesthesia, may lead to acute cardiac decompensation.
Cardiovascular Physiology & Anesthesia: Introduction
Anesthesiologists must have a thorough understanding of cardiovascular physiology both for its scientific significance in anesthesia and for its practical applications to patient management. Anesthetic successes and failures are often directly related to the skill of the practitioner in manipulating cardiovascular physiology. This chapter reviews the physiology of the heart and the systemic circulation and the pathophysiology of heart failure.
The circulatory system consists of the heart, blood vessels, and blood. Its function is to provide oxygen and nutrients to the tissues and to carry away the products of metabolism. The heart propels blood through two vascular systems arranged in series. In the normally low-pressure pulmonary circulation, venous blood flows past the alveolar-capillary membrane, takes up oxygen, and eliminates CO2. In the high pressure systemic circulation, oxygenated arterial blood is pumped to metabolizing tissues, and the by-products of metabolism are taken up for elimination by the lungs, kidneys, or liver.
The Heart
Although anatomically one organ, the heart can be functionally divided into right and left pumps, each consisting of an atrium and a ventricle. The atria serve as both conduits and priming pumps, whereas the ventricles act as the major pumping chambers. The right ventricle receives systemic venous (deoxygenated) blood and pumps it into the pulmonary circulation, whereas the left ventricle receives pulmonary venous (oxygenated) blood and pumps it into the systemic circulation. Four valves normally ensure unidirectional flow through each chamber. The normal pumping action of the heart is the result of a complex series of electrically driven and mechanical events. Electrical events precede mechanical ones.
The heart consists of specialized striated muscle in a connective tissue skeleton. Cardiac muscle can be divided into atrial, ventricular, and specialized pacemaker and conducting cells. The self-excitatory nature of cardiac muscle cells and their unique organization allow the heart to function as a highly efficient pump. Serial low-resistance connections (intercalated disks) between individual myocardial cells allow the rapid and orderly spread of depolarization in each pumping chamber. Electrical activity readily spreads from one atrium to another and from one ventricle to another via specialized conduction pathways. The normal absence of direct connections between the atria and ventricles except through the atrioventricular (AV) node delays conduction and enables atrial contraction to prime the ventricle.
At rest, the myocardial cell membrane is nominally permeable to K+, but is relatively impermeable to Na+. A membrane-bound Na+-K+–adenosine triphosphatase (ATPase) concentrates K+ intracellularly in exchange for extrusion of Na+ out of the cell. Intracellular Na+ concentration is kept low, whereas intracellular K+ concentration is kept high relative to the extracellular space. The relative impermeability of the membrane to calcium also maintains a high extracellular to cytoplasmic calcium gradient. Movement of K+ out of the cell and down its concentration gradient results in a net loss of positive charges from inside the cell. An electrical potential is established across the cell membrane, with the inside of the cell negative with respect to the extracellular environment, because anions do not accompany K+. Thus, the resting membrane potential represents the balance between two opposing forces: the movement of K+ down its concentration gradient and the electrical attraction of the negatively charged intracellular space for the positively charged potassium ions.
The normal ventricular cell resting membrane potential is −80 to −90 mV. As with other excitable tissues (nerve and skeletal muscle), when the cell membrane potential becomes less negative and reaches a threshold value, a characteristic action potential (depolarization) develops (Figure 20-1 and Table 20-1). The action potential transiently raises the membrane potential of the myocardial cell to +20 mV. In contrast to action potentials in axons, the spike in cardiac action potentials is followed by a plateau phase that lasts 0.2-0.3 sec. Whereas the action potential for skeletal muscle and nerves is due to the abrupt opening of voltage-gated sodium channels in the cell membrane, in cardiac muscle, it is initiated by voltage-gated sodium channels (the spike) and maintained by voltage-gated calcium channels (the plateau). Depolarization is also accompanied by a transient decrease in potassium permeability. Subsequent restoration of normal potassium permeability and termination of sodium and calcium channel permeability eventually restores the membrane potential to its resting value.
Figure 20-1
Cardiac action potentials. A: Note the characteristic contours of action potentials recorded from different parts of the heart. B: Pacemaker cells in the sinoatrial (SA) node lack the same distinct phases as atrial and ventricular muscle cells and display prominent spontaneous diastolic depolarization. See Table 20-1 for an explanation of the different phases of the action potential. (Modified and reproduced, with permission, from Barrett KE: Ganong’s Review of Medical Physiology, 24th ed., McGraw-Hill, 2012.)
Phase | Name | Event | Cellular Ion Movement |
---|---|---|---|
0 | Upstroke | Activation (opening) of voltage-gated Na+ channels | Na+ entry and decreased permeability to K+ |
1 | Early rapid repolarization | Inactivation of Na+ channel and transient increase in K+ permeability | K+ out (ITo) |
2 | Plateau | Activation of slow calcium channels | Ca2+ entry |
3 | Final repolarization | Inactivation of calcium channels and increased permeability to K+ | K+ out |
4 | Resting potential | Normal permeability restored (atrial and ventricular cells) | Na+-K+-ATPase pumps K+ in and Na+ out |
Diastolic repolarization | Intrinsic slow leakage of Ca2+ into cells that spontaneously depolarize | Ca2+ in |
Following depolarization, the cells are typically refractory to subsequent normal depolarizing stimuli until “phase 4.” The effective refractory period is the minimum interval between two depolarizing impulses that will propagate. In fast-conducting myocardial cells, this period is generally closely correlated with the duration of the action potential. In contrast, the effective refractory period in more slowly conducting myocardial cells can outlast the duration of the action potential.
Table 20-2 lists some of the multiple types of ion channels in cardiac muscle membrane. Some are activated by a change in cell membrane voltage, whereas others open only when bound by ligands. T-type (transient) voltage-gated calcium channels play a role in phase 0 of depolarization. During the plateau phase (phase 2), Ca2+ inflow occurs through slow L-type (long-lasting), voltage-gated calcium channels. Three major types of potassium channels are responsible for repolarization. The first results in a transient outward K+ current (ITo), the second is responsible for a short rectifying current (IKr), and the third produces a slowly acting rectifying current (IKs) that helps to restore the cell membrane potential to its resting value.
The cardiac impulse normally originates in the sinoatrial (SA) node, a group of specialized pacemaker cells in the sulcus terminalis, located posteriorly at the junction of the right atrium and the superior vena cava. These cells seem to have an outer membrane that leaks Na+ (and possibly Ca2+). The slow influx of Na+, which results in a less negative resting membrane potential (−50 to −60 mV), has three important consequences: near constant inactivation of voltage-gated sodium channels, an action potential with a threshold of −40 mV that is primarily due to ion movement across the slow calcium channels, and regular spontaneous depolarizations. During each cycle, intracellular leakage of Na+ causes the cell membrane to become progressively less negative; when the threshold potential is reached, calcium channels open, K+ permeability decreases, and an action potential develops. Restoration of normal K+ permeability returns the cells in the SA node to their normal resting membrane potential.
The impulse generated at the SA node is normally rapidly conducted across the atria and to the AV node. Specialized atrial fibers may speed up conduction to both the left atrium and the AV node. The AV node, which is located in the septal wall of the right atrium, just anterior to the opening of the coronary sinus and above the insertion of the septal leaflet of the tricuspid valve, is actually made up of three distinct areas: an upper junctional (AN) region, a middle nodal (N) region, and a lower junctional (NH) region. Although the N region does not possess intrinsic spontaneous activity (automaticity), both junctional areas do. The normally slower rate of spontaneous depolarization in AV junctional areas (40-60 times/min) allows the faster SA node to control heart rate. Any factor that decreases the rate of SA node depolarization or increases the automaticity of AV junctional areas allows the junctional areas to function as the pacemaker for the heart.
Impulses from the SA node normally reach the AV node after about 0.04 sec, but leave after another 0.11 sec. This delay is the result of the slowly conducting small myocardial fibers within the AV node, which depend on slow calcium channels for propagation of the action potential. In contrast, conduction of the impulse between adjoining cells in the atria and in the ventricles is due primarily to activation of sodium channels. The lower fibers of the AV node combine to form the common bundle of His. This specialized group of fibers passes into the interventricular septum before dividing into left and right branches to form the complex network of Purkinje fibers that depolarizes both ventricles. In sharp contrast to AV nodal tissue, His-Purkinje fibers have the fastest conduction velocities in the heart, resulting in nearly simultaneous depolarization of the entire endocardium of both ventricles (normally within 0.03 s). Synchronized depolarization of the lateral and septal walls of the left ventricle promotes effective ventricular contraction. The spread of the impulse from the endocardium to the epicardium through ventricular muscle requires an additional 0.03 sec. Thus, an impulse arising from the SA node normally requires less than 0.2 sec to depolarize the entire heart.
Potent inhaled anesthetics depress SA node automaticity. These agents seem to have only modest direct effects on the AV node, prolonging conduction time and increasing refractoriness. This combination of effects likely explains the occurrence of junctional tachycardia when an anticholinergic is administered for sinus bradycardia during inhalation anesthesia; junctional pacemakers are accelerated more than those in the SA node. The electrophysiological effects of volatile agents on Purkinje fibers and ventricular muscle are complex due to autonomic interactions. Both antiarrhythmic and arrhythmogenic properties are described. The former may be due to direct depression of Ca2+ influxes, whereas the latter generally involves potentiation of catecholamines, especially with halothane. The arrhythmogenic effect requires activation of both α1– and β-adrenergic receptors. Intravenous induction agents have limited electrophysiological effects in usual clinical doses. Opioids, particularly fentanyl and sufentanil, can depress cardiac conduction, increasing AV node conduction and the refractory period and prolonging the duration of the Purkinje fiber action potential.
Local anesthetics have important electrophysiological effects on the heart at blood concentrations that are generally associated with systemic toxicity. In the case of lidocaine, electrophysiological effects at low blood concentrations can be therapeutic. At high blood concentrations, local anesthetics depress conduction by binding to sodium channels; at extremely high concentrations, they also depress the SA node. The most potent local anesthetics—bupivacaine, etidocaine, and to a lesser degree, ropivacaine—seem to have the most potent effects on the heart, particularly on Purkinje fibers and ventricular muscle. Bupivacaine binds open or inactivated sodium channels and dissociates from them slowly. It can cause profound sinus bradycardia and sinus node arrest and malignant ventricular arrhythmias; furthermore, it can depress left ventricular contractility. Twenty percent lipid emulsions have been used to treat local anesthetic cardiac toxicity. The mechanisms of action of this therapy are unclear, although possibilities include serving as a lipid reservoir and decreasing lipophilic toxic local anesthetics in the myocardium.
Calcium channel blockers are organic compounds that block Ca2+ influx through L-type but not T-type channels. Dihydropyridine blockers, such as nifedipine, simply plug the channel, whereas other agents, such as verapamil, and to a lesser extent, diltiazem, preferentially bind the channel in its depolarized inactivated state (use-dependent blockade).
Myocardial cells contract as a result of the interaction of two overlapping, rigid contractile proteins, actin and myosin. These proteins are fixed in position within each cell during both contraction and relaxation. Dystrophin, a large intracellular protein, connects actin to the cell membrane (sarcolemma). Cell shortening occurs when the actin and myosin are allowed to fully interact and slide over one another. This interaction is normally prevented by two regulatory proteins, troponin and tropomyosin; troponin is composed of three subunits (troponin I, troponin C, and troponin T). Troponin is attached to actin at regular intervals, whereas tropomyosin lies within the center of the actin structure. An increase in intracellular Ca2+ concentration (from about 10-7 to 10-5 mol/L) promotes contraction as Ca2+ ions bind troponin C. The resulting conformational change in these regulatory proteins exposes the active sites on actin that allow interaction with myosin bridges (points of overlapping). The active site on myosin functions as a magnesium-dependent ATPase whose activity is enhanced by the increase in intracellular Ca2+ concentration. A series of attachments and disengagements occur as each myosin bridge advances over successive active sites on actin. Adenosine triphosphate (ATP) is consumed during each attachment. Relaxation occurs as Ca2+ is actively pumped back into the sarcoplasmic reticulum by a Ca2+-Mg2+-ATPase; the resulting drop in intracellular Ca2+ concentration allows the troponin-tropomyosin complex to again prevent the interaction between actin and myosin.
The quantity of Ca2+ ions required to initiate contraction exceeds that entering the cell through slow calcium channels during phase 2. The small amount that does enter through slow calcium channels triggers the release of much larger amounts of Ca2+ stored intracellularly (calcium-dependent calcium release) within cisterns in the sarcoplasmic reticulum.
The action potential of muscle cells depolarizes their T systems, tubular extensions of the cell membrane that transverse the cell in close approximation to the muscle fibrils, via dihydropyridine receptors (voltage-gated calcium channels). This initial increase in intracellular Ca2+ triggers an even greater Ca2+ inflow across ryanodine receptors, a nonvoltage- dependent calcium channel in the sarcoplasmic reticulum. The force of contraction is directly dependent on the magnitude of the initial Ca2+ influx. During relaxation, when the slow channels close, a membrane-bound ATPase actively transports Ca2+ back into the sarcoplasmic reticulum. Ca2+ is also extruded extracellularly by an exchange of intracellular Ca2+ for extracellular sodium by an ATPase in the cell membrane. Thus, relaxation of the heart also requires ATP.
The quantity of intracellular Ca2+ available, its rate of delivery, and its rate of removal determine, respectively, the maximum tension developed, the rate of contraction, and the rate of relaxation. Sympathetic stimulation increases the force of contraction by raising intracellular Ca2+ concentration via a β1-adrenergic receptor-mediated increase in intracellular cyclic adenosine monophosphate (cAMP) through the action of a stimulatory G protein. The increase in cAMP recruits additional open calcium channels. Moreover, adrenergic agonists enhance the rate of relaxation by enhancing Ca2+ reuptake by the sarcoplasmic reticulum. Phosphodiesterase inhibitors, such as inamrinone, enoximone, and milrinone, produce similar effects by preventing the breakdown of intracellular cAMP. Digitalis glycosides increase intracellular Ca2+ concentration through inhibition of the membrane-bound Na+-K+-ATPase; the resulting small increase in intracellular Na+ allows for a greater influx of Ca2+ via the Na+-Ca2+ exchange mechanism. Glucagon enhances contractility by increasing intracellular cAMP levels via activation of a specific nonadrenergic receptor. The new agent levosimendan is a calcium sensitizer that enhances contractility by binding to troponin C. In contrast, release of acetylcholine following vagal stimulation depresses contractility through increased cyclic guanosine monophosphate (cGMP) levels and inhibition of adenylyl cyclase; these effects are mediated by an inhibitory G protein. Acidosis blocks slow calcium channels and therefore also depresses cardiac contractility by unfavorably altering intracellular Ca2+ kinetics.
Studies suggest that volatile anesthetics depress cardiac contractility by decreasing the entry of Ca2+ into cells during depolarization (affecting T- and L-type calcium channels), altering the kinetics of its release and uptake into the sarcoplasmic reticulum, and decreasing the sensitivity of contractile proteins to Ca2+. Halothane and enflurane seem to depress contractility more than isoflurane, sevoflurane, and desflurane. Anesthetic-induced cardiac depression is potentiated by hypocalcemia, β-adrenergic blockade, and calcium channel blockers. Nitrous oxide also produces concentration-dependent decreases in contractility by reducing the availability of intracellular Ca2+ during contraction. The mechanisms of direct cardiac depression from intravenous anesthetics are not well established, but presumably involve similar actions. Of all the major intravenous induction agents, ketamine seems to have the least direct depressant effect on contractility. Local anesthetic agents also depress cardiac contractility by reducing Ca2+ influx and release in a dose-dependent fashion. The more potent (at nerve block) agents, such as bupivacaine, tetracaine, and ropivacaine, more significantly depress left ventricular contractility than less potent (at nerve block) agents, such as lidocaine or chloroprocaine.
Parasympathetic fibers primarily innervate the atria and conducting tissues. Acetylcholine acts on specific cardiac muscarinic receptors (M2) to produce negative chronotropic, dromotropic, and inotropic effects. In contrast, sympathetic fibers are more widely distributed throughout the heart. Cardiac sympathetic fibers originate in the thoracic spinal cord (T1-T4) and travel to the heart initially through the cervical (stellate) ganglia and from the ganglia as the cardiac nerves. Norepinephrine release causes positive chronotropic, dromotropic, and inotropic effects primarily through activation of β1-adrenergic receptors. β2-Adrenergic receptors are normally fewer in number and are found primarily in the atria; activation increases heart rate and, to a lesser extent, contractility.
Cardiac autonomic innervation has an apparent sidedness, because the right sympathetic and right vagus nerves primarily affect the SA node, whereas the left sympathetic and vagus nerves principally affect the AV node. Vagal effects frequently have a very rapid onset and resolution, whereas sympathetic influences generally have a more gradual onset and dissipation. Sinus arrhythmia is a cyclic variation in heart rate that corresponds to respiration (increasing with inspiration and decreasing during expiration); it is due to cyclic changes in vagal tone.
The cardiac cycle can be defined by both electrical and mechanical events (Figure 20-2). Systole refers to contraction and diastole refers to relaxation. Most diastolic ventricular filling occurs passively before atrial contraction. Contraction of the atria normally contributes 20% to 30% of ventricular filling. Three waves can generally be identified on atrial pressure tracings (Figure 20-2). The a wave is due to atrial systole. The c wave coincides with ventricular contraction and is said to be caused by bulging of the AV valve into the atrium. The v wave is the result of pressure buildup from venous return before the AV valve opens again. The x descent is the decline in pressure between the c and v waves and is thought to be due to a pulling down of the atrium by ventricular contraction. Incompetence of the AV valve on either side of the heart abolishes the x descent on that side, resulting in a prominent cv wave. The y descent follows the v wave and represents the decline in atrial pressure as the AV valve opens. The notch in the aortic pressure tracing is referred to as the incisura and is said to represent the brief pressure change from transient backflow of blood into the left ventricle just before aortic valve closure.
Discussions of ventricular function usually refer to the left ventricle, but the same concepts apply to the right ventricle. Although the ventricles are often thought of as functioning separately, their interdependence has clearly been demonstrated. Moreover, factors affecting systolic and diastolic functions can be differentiated: Systolic function involves ventricular ejection, whereas diastolic function is related to ventricular filling.
Ventricular systolic function is often (erroneously) equated with cardiac output, which can be defined as the volume of blood pumped by the heart per minute. Because the two ventricles function in series, their outputs are normally equal. Cardiac output (CO) is expressed by the following equation:
CO = SV × HR
where SV is the stroke volume (the volume pumped per contraction) and HR is heart rate. To compensate for variations in body size, CO is often expressed in terms of total body surface area:
where CI is the cardiac index and BSA is body surface area. BSA is usually obtained from nomograms based on height and weight (Figure 20-3). Normal CI is 2.5-4.2 L/min/m2. Because the normal CI has a wide range, it is a relatively insensitive measurement of ventricular performance. Abnormalities in CI therefore usually reflect gross ventricular impairment. A more accurate assessment can be obtained if the response of the cardiac output to exercise is evaluated. Under these conditions, failure of the cardiac output to increase and keep up with oxygen consumption is reflected by a decreasing mixed venous oxygen saturation. A decrease in mixed venous oxygen saturation in response to increased demand usually reflects inadequate tissue perfusion.
Thus, in the absence of hypoxia or severe anemia, measurement of mixed venous oxygen tension (or saturation) is an excellent estimate of the adequacy of cardiac output.
When stroke volume remains constant, cardiac output is directly proportional to heart rate. Heart rate is an intrinsic function of the SA node (spontaneous depolarization), but is modified by autonomic, humoral, and local factors. The normal intrinsic rate of the SA node in young adults is about 90-100 beats/min, but it decreases with age based on the following formula:
Normal intrinsic heart rate = 118 beats/min – (0.57 × age)
Enhanced vagal activity slows the heart rate via stimulation of M2 cholinergic receptors, whereas enhanced sympathetic activity increases the heart rate mainly through activation of β1-adrenergic receptors and, to lesser extent, β2-adrenergic receptors (see above).
Stroke volume is normally determined by three major factors: preload, afterload, and contractility. This analysis is analogous to laboratory observations on skeletal muscle preparations. Preload is muscle length prior to contraction, whereas afterload is the tension against which the muscle must contract. Contractility is an intrinsic property of the muscle that is related to the force of contraction but is independent of both preload and afterload. Because the heart is a three-dimensional multichambered pump, both ventricular geometric form and valvular dysfunction can also affect stroke volume (Table 20-3).
Ventricular preload is end-diastolic volume, which is generally dependent on ventricular filling. The relationship between cardiac output and left ventricular end-diastolic volume is known as Starling’s law of the heart (Figure 20-4). Note that when the heart rate and contractility remain constant, cardiac output is directly proportional to preload until excessive end-diastolic volumes are reached. At that point, cardiac output does not appreciably change—or may even decrease. Excessive distention of either ventricle can lead to excessive dilatation and incompetence of the AV valves.
Ventricular filling can be influenced by a variety of factors (Table 20-4), of which the most important is venous return. Because most of the other factors affecting venous return are usually fixed, vascular capacity is normally its major determinant. Increases in metabolic activity reduce vascular capacity, so that venous return to the heart increases as the volume of venous capacitance vessels decreases. Changes in blood volume and venous tone are important causes of intraoperative and postoperative changes in ventricular filling and cardiac output. Any factor that alters the normally small venous pressure gradient favoring blood return to the heart also affects cardiac filling. Such factors include changes in intrathoracic pressure (positive-pressure ventilation or thoracotomy), posture (positioning during surgery), and pericardial pressure (pericardial disease).
The most important determinant of right ventricular preload is venous return. In the absence of significant pulmonary or right ventricular dysfunction, venous return is also the major determinant of left ventricular preload
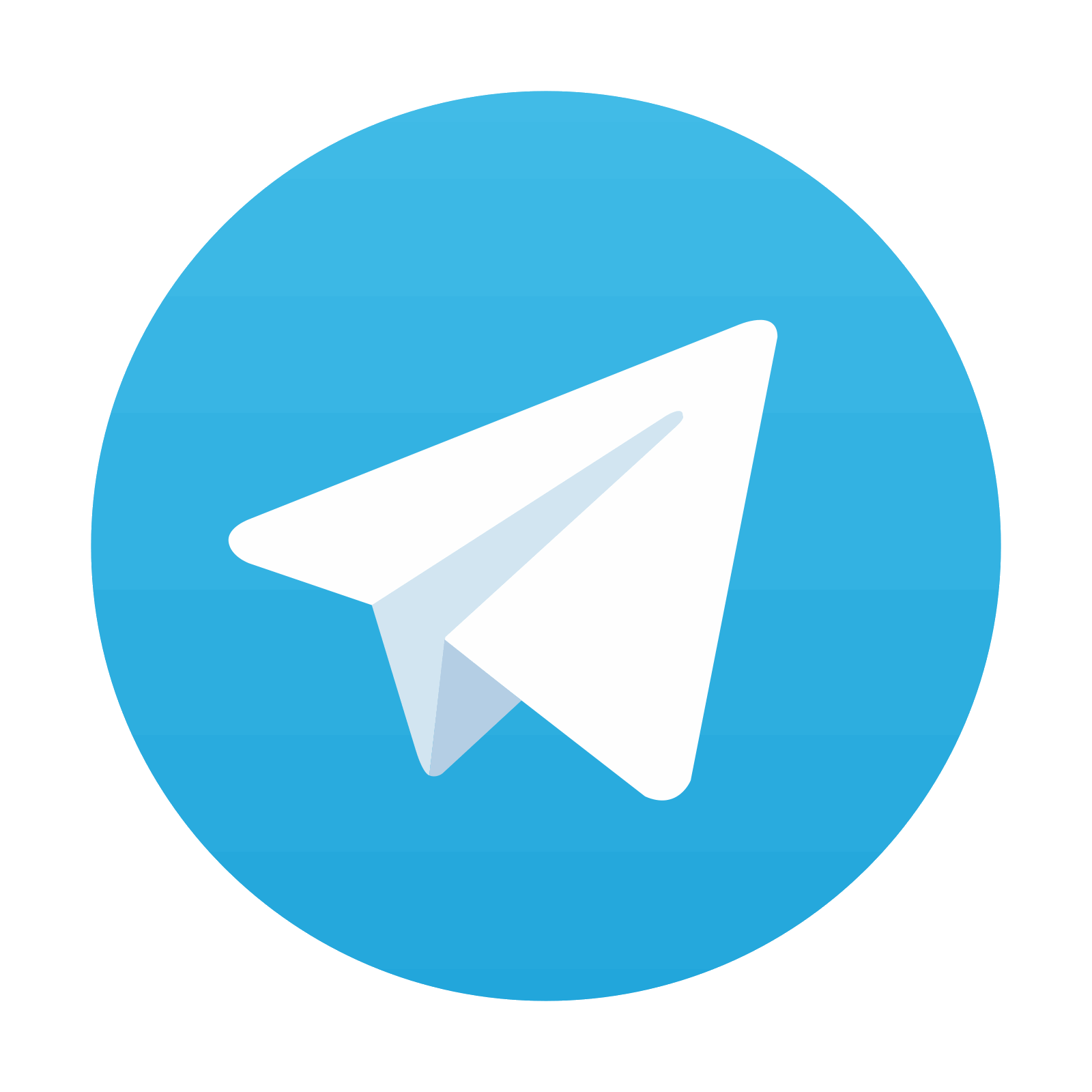
Stay updated, free articles. Join our Telegram channel
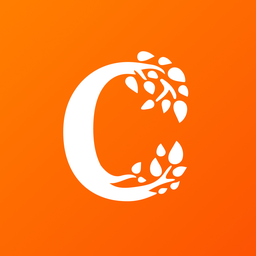
Full access? Get Clinical Tree
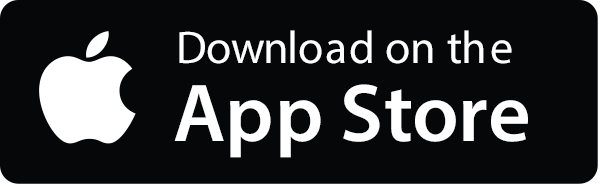
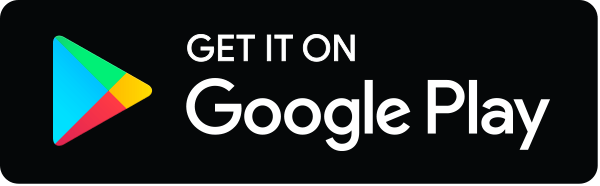
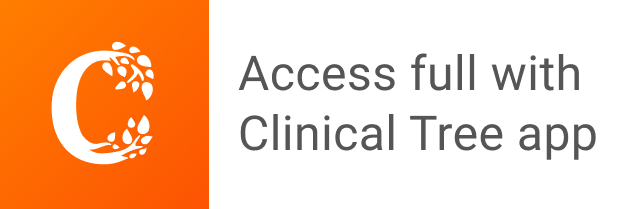