SEPSIS
DEFINITION
Sepsis is a common cause of morbidity and mortality in the surgical intensive care unit (SICU) accounting for an estimated 700,000 cases per year in the United States.1 Sepsis is defined by the presence of infection in addition to a systemic inflammatory response syndrome (SIRS). Clinically, SIRS can manifest as fever, leukocytosis, tachycardia, and/or tachypnea. Severe sepsis is defined as sepsis with concomitant organ dysfunction. Septic shock is characterized by sepsis with persistent hypotension (systolic blood pressure < 90 mm Hg or mean arterial pressure [MAP] < 60) despite volume resuscitation.2 Sepsis is currently the 10th most common cause of death in the United States, accounting for over 34,000 deaths per year.3 Mortality rates for patients admitted with sepsis approach 20%-30%, with increased mortality seen in the nonwhite population and the elderly.1
PATHOPHYSIOLOGY
Sepsis occurs as a result of a complex set of interactions, incited by an invading pathogen, which result in a substantial host inflammatory response and activation of both the complement and coagulation cascades. While these responses are essential to control and eradicate the infecting microorganism, they are also capable of causing major damage to host tissues.
MICROBIAL FACTORS
Gram-positive bacteria, gram-negative bacteria, fungi, and parasites are capable of causing sepsis. Gram-negative bacteria have a cell wall that contains lipopolysaccharide (LPS) on its outer layer. The pathogenicity of gram-negative bacteria is due to LPS, which elicits an innate immune response. Extensive studies using animal models have characterized the ability of LPS to cause a significant host inflammatory response, with pathophysiologic responses similar to those seen in humans with sepsis.4 Studies in human volunteers have further confirmed the toxicity of endotoxin itself, showing that injection of small concentrations of LPS causes fever, activation of inflammatory cells, and increased cytokine production.5
Until recently, gram-negative bacteria were the most common cause of sepsis in the ICU. There has been an increase in the incidence of gram-positive bacteria causing severe sepsis, now identified as the causative bacteria in up to 50% of the cases.6 Gram-positive bacteria exert their pathogenic effects through the release of exotoxins and contain an outer polysaccharide capsule that protects against phagocytosis. Gram-positive bacteria contain a thick peptidoglycan layer that forms the cell wall. It is the peptidoglycan layer of gram-positive bacteria that is recognized by inflammatory cells of the innate immune system. Fungi remain a rare cause of severe sepsis, causing approximately 5% of cases.
INFLAMMATORY RESPONSE
The innate immune system is responsible for recognizing an invading microorganism. The innate immune system consists of peripheral blood mononuclear cells and polymorphonuclear leukocytes (PMNs). These cells recognize pathogen-associated molecular patterns (PAMPs), which are displayed by gram-positive bacteria, gram-negative bacteria, fungi, parasites, and viruses. Sepsis also results in the release of damage-associated molecular patterns (DAMPs), which are released by damaged or dying host tissues. The innate immune system initiates an inflammatory response when these PAMPs and DAMPs bind to pattern recognition receptors on host inflammatory cells.7 In sepsis, there are high levels of DAMPs and PAMPs released, which can cause an exaggerated response from inflammatory cells, resulting in damage to host tissues.8
The toll-like receptor (TLR) family is an example of pattern recognition receptors that are key mediators of this inflammatory response. TLR expression is up-regulated, and TLR receptor reactivity is enhanced in patients with sepsis.9 TLR-4 has been shown to be the receptor that binds to LPS, triggering the activation of multiple inflammatory signaling cascades.10,11 The importance of the TLR-4 receptor in sepsis has been well documented in animal models using TLR-4 knockout and mutant mice.12 These studies have shown that mice with mutations in their TLR4 gene exhibit endotoxin tolerance and are unable to mount an inflammatory response upon exposure to LPS.13
Binding of a PAMP to the TLR receptor activates numerous intracellular signaling pathways that up-regulate the production of transcriptional factors. These transcriptional factors, including nuclear factor-kappa B (NF-KB), activator protein-1 (AP-1), and phosphoinositide 3-kinase (PI3K)/Akt, activate the inflammatory response resulting in the production of cytokines, acute phase proteins, and inducible nitric oxide synthase (iNOS). Proinflammatory cytokines such as tumor necrosis factor-alpha (TNF-α) and interleukin (IL)-1β exert several downstream effects that propagate the inflammatory response. Studies in animal models of sepsis have shown that there is a relationship between increased TNF-α production and the development of organ failure and septic shock.14 TNF-α exerts these effects through the production of other proinflammatory mediators such as leukotrienes and prostaglandins, which together mediate the inflammatory response in sepsis by increasing capillary leak, causing damage to epithelial and endothelial barriers and increasing adhesion molecules on both inflammatory cells and vascular endothelial cells. The PMN is the primary effector cell that mediates the acute inflammatory response to invading microorganisms. Activated PMNs bind to adhesion molecules expressed on endothelial cells allowing for diapedesis into the tissues. There, PMNs respond to the pathogen through phagocytosis, degranulation, and oxidative burst. Oxidative burst results in the release of reactive oxygen species (ROS), which leads to bacterial killing. While PMN activation and degranulation is essential in neutralizing the invading pathogen, the release of ROS can also be deleterious to the host tissues, resulting in organ injury.
The complement cascade is a part of the innate immune system that responds to invading pathogens. The complement system is composed of circulating plasma proteins that are normally in their inactive state. This system is highly regulated, as activation of the complement cascade can be damaging to host tissues. Once activated by a stimulus such as the presence of microorganisms, proteins of the complement system become activated. These activated complement proteins are responsible for lysis of bacteria and viruses, and for opsonization of bacteria resulting in phagocytosis by other inflammatory cells.
The complement cascade can be activated through three different pathways.15 In the classic pathway, complement proteins are activated by immunoglobulin-G (IgG) or IgM bound to an antigen. The classic pathway is also activated by acute phase proteins that are produced in response to invading pathogens. The alternate pathway of complement activation occurs when complement binds directly to the PAMPs displayed by bacteria, viruses, and fungi and does not rely on antibodies binding to the pathogen. The mannose-binding lectin pathway is similar to the classic pathway of complement activation. Mannose-binding lectin is produced by the liver in response to infection and binds to the surface of invading pathogens, initiating the complement cascade. Activation of the complement system also alters the coagulation cascade, linking these two pathways in the response to sepsis.
COAGULATION
Sepsis increases the procoagulant balance in the host resulting in increased risk of small vessel thrombosis, decreased microcirculatory blood flow, and possible tissue ischemia. Inflammation is a potent activator of tissue factor that can initiate the coagulation cascade.16 Activated tissue factor complexes with factor VIIa ultimately resulting in the conversion of prothrombin to thrombin. Thrombin then converts fibrinogen into fibrin leading to the formation of thrombus. The importance of sepsis-induced tissue factor activation was demonstrated in a study by de Jonge et al.17 in which a tissue factor pathway inhibitor decreased coagulation activation after exposure to endotoxin. Sepsis also decreased the presence of anticoagulant factors in the blood, further shifting the procoagulant balance. The effects of activated protein C, antithrombin III, and the tissue factor pathway inhibitor are diminished in response to inflammation.
Disseminated intravascular coagulation (DIC) is a frequent complication in septic patients. DIC is characterized by the activation of the coagulation cascade resulting in fibrin activation and microvascular thrombosis, which is accompanied by consumption of coagulation factors and platelets.18 Decreased microcirculatory blood flow caused by microvascular thrombosis can result in tissue ischemia and organ failure. Consumption of coagulation factors and platelets puts patients at risk for serious bleeding complications and may require transfusion of blood products. In a bleeding patient with DIC, platelets should be maintained at >20-30 × 10,9 cryoprecipitate given to maintain fibrinogen concentration > 100 mg per dL, and fresh frozen plasma given to correct the PT and PTT.19 Replacement therapy may be needed repeatedly until the underlying cause of DIC is treated as consumption of blood products will continue. Treatment of DIC is focused on treating the source of sepsis, as DIC typically resolves upon treatment of the underlying cause of infection.
MULTIPLE ORGAN FAILURE
The exaggerated host response to the septic insult can lead to major tissue injury, end organ dysfunction, and ultimately multiple organ failure (MOF). In fact, mortality rates in critically ill septic patients have been shown to be related to the magnitude of the host inflammatory response, rather than to the type of bacteria responsible for the infection or the extent of the infectious insult.20 MOF is an important cause of mortality in critically ill patients, with mortality rates directly related to the number of organ systems that have failed. ICU patients who develop MOF have a 20-fold increased mortality compared to ICU patients who do not develop MOF, as well as a significantly increased ICU and hospital length of stay.21 Therefore, the timely diagnosis and prompt treatment of the septic patient is essential for improved outcomes in this population.
DIAGNOSIS OF SEPSIS
Patients suspected to have sepsis should be monitored closely for signs of the SIRS response and evidence of end organ dysfunction. Prompt identification of the source of infection is a cornerstone in the treatment of sepsis. It is critically important to obtain specimens for culture including blood, urine, sputum, peritoneal fluid, abscess drainage, and catheter tips. It is vital that these cultures be obtained prior to beginning broad-spectrum antibiotic therapy. Culture specimens obtained following administration of antibiotics may prevent the identification of the organism responsible for the septic insult and later limit the ability of the clinician to properly tailor antimicrobial therapy. It is important to note that a clear microbiologic source of sepsis is not identified through cultures in at least one-third of cases.22 Prompt imaging is also important in the diagnosis of the septic source, including plain radiographs and CT scans. Imaging studies should be chosen based on the clinical presentation and the presumed source of infection.
Identification of laboratory markers of sepsis or surrogates to measure the response of the septic patient is the topic of considerable research efforts. Biomarkers may play several roles in the clinical setting, including identification of patients at risk for an adverse outcome related to sepsis, to diagnose sepsis and inform treatment decisions, to rule out sepsis, for risk stratification, to monitor the response to treatment, and for use as a surrogate end point to monitor the effect of various treatment strategies.23 The diverse host response to microbial infection and sepsis make identification of clinically reliable markers quite difficult.
Currently biomarkers are not widely used in the clinical setting to diagnose or guide the treatment of the septic patient. While hundreds of unique biomarkers have been proposed as possible markers of sepsis, a few potentially promising candidates have been studied. C-reactive protein (CRP) has been used for several years in an attempt to guide the management of septic patients. CRP has long been used as a marker of generalized inflammation. CRP levels increase within 6 hours of the onset of infection, with CRP levels doubling every 8 hours as the infection persists. CRP levels have been shown to peak near 36 hours after the onset of infection, with levels 1,000 times higher than normal physiologic levels.24 CRP levels that remain persistently elevated in patients with sepsis are associated with worse outcomes.25 CRP levels may help guide the effectiveness of antibiotic therapy in the septic patient, with decreasing CRP levels of the first 48 hours indicating effective antimicrobial therapy.26
Procalcitonin (PCT) is a precursor molecule of calcitonin, which has been shown to be released in response to inflammation and infection. Recent studies suggest that PCT may be a superior marker of inflammation compared to CRP.27 Increases in circulating PCT are related to the severity of sepsis, with PCT levels decreasing after institution of appropriate antibiotic therapy.28,29 In a study of healthy volunteers injected with endotoxin, PCT levels peaked within 6 hours and maintained a plateau between 8 and 24 hours after injection.30 Decreased PCT levels between days 2 and 3 are associated with improved survival and may represent a way to monitor the effectiveness of antibiotic therapy.31 Measurement of PCT may allow for the rapid identification of patients at risk for developing sepsis; however, further randomized control trials are needed to further define its clinical utility.
MANAGEMENT OF SEPSIS AND SEPTIC SHOCK
In the setting of acute organ dysfunction with or without hypotension, the speed and appropriateness of therapy are crucial to achieving treatment goals. In 2008, phase II of the Surviving Sepsis Campaign was published with clear guidelines for the treatment of sepsis and septic shock.32 Since publication of those guidelines, sepsis bundles have emerged to eliminate piecemeal application of the guidelines and to make it easier for clinicians to bring the guidelines into practice.33 In the surgical setting, life-threatening oxygenation, ventilation, and circulation deficits must be addressed quickly with complete interrogation of the patient for source of infection. Swift action with appropriate decision making is key in reducing mortality.
Specific treatment guidelines, protocols, and bundles have been shown to reduce morbidity and mortality of severe sepsis and septic shock in addition to reducing health care costs.34–37 Although no study has been designed to give evidence of a clear causal relationship between adherence to SSC guidelines and reduction in mortality, these observational studies do provide a strong association between reduced mortality and use of the guidelines, whether in bundle format or not. Levy et al.34 reported on the >15,000 patients registered in the SSC database and found a reduction in mortality from 37% to 30.8% over 2 years with use of SSC guidelines. Two additional studies demonstrate that compliance with a 6-hour bundle is significantly associated with an up to 50% reduction in mortality.35,37
Despite the attractive evidence supporting a benefit of the SSC, the guidelines that were produced have had their shortcomings. The initial goals of the campaign were to reduce the mortality related to sepsis by 25% over 5 years and provide a backbone for quality improvement in the treatment of severe sepsis and septic shock. Over the 5-year course, only a 20% reduction in mortality was achieved.38 Although commendable, this is still regarded as failure. The majority of studies report <50% compliance with all or a major portion of the guidelines. This illustrates the difficulty in producing a large global shift in management strategies. The education, implementation, mentoring, and compliance to new policies and procedures have countless obstacles, be it resources, funding, or agreement to change practice patterns. Finally, a causal relationship between the implementation of the guidelines and an establishment of the reduction in mortality must be made through firm research, likely prospective in nature.39
EARLY PHASE OF MANAGEMENT
Vital signs, central venous pressure, urinary output, and physical findings do not adequately assess tissue hypoxia in severe disease. Early goal-directed therapy gives specific resuscitation end points that give a more accurate picture of adequate tissue perfusion and oxygenation (Algorithm. 12.1). Resuscitation end points include not only global indicators of perfusion (i.e., heart rate, blood pressure, urine output) but also mixed venous oxygen saturation, arterial lactate concentration, based deficit, and pH.40 Specifically, within the first 6 hours of onset of severe sepsis or septic shock, intravascular volume should be increased to achieve CVP 8-12 mm Hg and vasopressors should be initiated for MAP < 65 mm Hg, vasodilators for MAP > 90 mm Hg. If central venous oxygen saturation measured within the superior vena cava or right atria is <70%, transfusion of packed red blood cells could be considered to achieve a hematocrit of ≥ 30%, especially in cases of active cardiac ischemia. If all these parameters are met and ScvO2 remains <70%, initiation of inotropic support was recommended. Identification of the source of infection and initiation of antibiotic therapy are also of utmost importance. Initiation of broad-spectrum antibiotics, preferably after multiple blood cultures have been obtained, is absolutely necessary as early as possible. Ideally, the source of infection is also identified and addressed within the first 6 hours of presentation. Use of and compliance with early goal-directed therapy produced a statistically significant increased achievement of resuscitation end points and significant reduction of in-hospital, 28-, and 60-day mortality.40
ALGORITHM 12.1 This chart represents the specific goals and indicators as an example of how to manage the septic patient upon presentation.
There has been much debate over the use of crystalloid or colloid to expand intravascular volume in hypotensive, septic patients. Two large meta-analyses produced differing results in choice of crystalloid versus colloid. Choi et al.41 found no difference with respect to mortality and pulmonary edema. However, subgroup analysis in trauma patients did seem to favor crystalloid over colloid. Schierhout and Roberts42 concluded a 4% increased risk of mortality when using colloid over crystalloid for initial resuscitation. One study attempted to measure the distribution of 5% albumin versus normal saline in the treatment of critically ill patients. Ultimately, through specific scientific measurements, the change in extracellular fluid volume to include both the plasma volume (intravascular) and the interstitial volume (edema) was expanded relatively equally per given MAP.43 Although nearly three times more normal saline was needed to achieve that of 5% albumin, the cost benefit greatly favored normal saline. The SAFE trial demonstrates equivalent outcomes of either 4% albumin or normal saline in the resuscitation of critically ill patients in the ICU.44
The 2008 Surviving Sepsis Campaign recommends using fluid challenges to achieve an adequate cardiac filling pressure while still affecting change in overall hemodynamics. Once hemodynamic improvement is lost, fluid administration should be slowed. Overall, there is no clear consensus whether colloid or crystalloid is superior over the other. Clinicians must use good judgement in making individual assessments based on patient needs and severity of disease.
OXYGEN DELIVERY
The state of sepsis on a systemic level is a mismatch of oxygen delivery with oxygen extraction. The combination of the two leads to an oxygen debt within tissues. Oxygen delivery can be manipulated in several ways, ultimately leaving the disorder of tissue oxygen extraction in inflammatory states as the root of organ dysfunction. As the inflammatory cascade progresses leading to vascular permeability and tissue edema, oxygen extraction only becomes more difficult. Early goal-directed therapy has a clear algorithm for increasing oxygen delivery. Initially, volume resuscitation is stressed, followed by vasoactive agents with or without blood transfusion to achieve the desired ScvO2 of ≥ 70%, and finally adding inotropic agents. The Surviving Sepsis Campaign advocates either blood transfusion to Hct ≥ 30% or adding inotropic agents to achieve goal ScvO2 ≥ 70%. Hébert et al.45 demonstrated that a restrictive transfusion strategy to maintain hemoglobin concentration between 7 and 9 g per dL was associated with significantly reduced in-hospital, 30-day, and 60-day mortality compared to liberal strategy between 10 and 12 g per dL. With the exception of a subset population of patients with active ongoing cardiac ischemia, these recommendations applied to critically ill patients once euvolemia was obtained with initial resuscitation. With respect to transfusion of other human blood products, transfusion of fresh frozen plasma to correct coagulopathy should be based on circumstances in which the patient is actively bleeding or in anticipation of surgery or procedure. Platelet transfusion is indicated when counts are <5,000 per mm3 regardless of lack of ongoing bleeding, considered when counts are between 5,000 and 30,000 per mm3, and to achieve goal of ≥ 50,000 for invasive procedure.32
In conjunction with raising the oxygen content of blood via transfusion, the second component of increasing oxygen delivery is affecting cardiac output after achieving a target MAP of ≥65 mm Hg. The SSC recommends either dopamine or norepinephrine as first-line vasopressor support for septic patients with hypotension. However, dopamine is favored as the vasopressor of choice if there is no contraindication.32 Physiologic dosage of vasopressin (0.03 U per minute) may be added as an adjunct with expectation that vasopressin will have the same effect as norepinephrine alone. In the setting of low ScvO2 after adequate fluid resuscitation, MAP ≥ 65 mm Hg, and if adequate hematocrit has been achieved, the use of an inotropic agent is recommended.40,46 There has been debate that increasing cardiac index to levels above the normal maximal average, the “supranormal” range, may have benefit by restoring hemostasis sooner and repaying the oxygen debt. Two randomized, controlled trials, however, failed to show a benefit in mortality with supranormal cardiac indices.46,47 In fact, a study Hayes et al. was terminated prematurely due to an increase in mortality in patients achieving supranormal physiologic conditions.
SOURCE CONTROL AND ANTIBIOTICS
Admissions to the SICU with the diagnosis of sepsis often result from specific infection. In the United States, the most common sites of infection are the respiratory tract (56.8%), the bloodstream (26.9%), the renal/urinary tract (22.2%), and the abdomen (16.6%).48 Many of these infections require surgical or invasive intervention to control the source of infection quickly. Pathologies such as necrotizing fasciitis, ascending cholangitis, closed-loop bowel obstruction, or intestinal perforation can be treated quickly and not necessarily definitively and still have a major impact on morbidity and mortality. Often, a lower physiologic insult is the more appropriate choice. SSC guidelines recommend that source control be achieved within 6 hours of admission to the hospital/ICU.32 For example, if available, percutaneous transhepatic cholangiography with drain placement by an interventional radiologist may be more appropriate and less stressful on a patient than operative choledochotomy for the treatment of cholangitis.
Line sepsis is a not uncommon consequence of prolonged ICU stay and can often be difficult to diagnose. Lines are frequently empirically removed or exchanged over guidewire to eliminate them as the source of infection. Older methods of blood culture often did not yield an expeditious answer and would frequently take days to determine if the indwelling catheter was truly the source of infection. New developments in laboratory technology can now provide automatic detection of microbial growth in blood cultures and measure the time elapsed between bottle inoculation and time to positivity. These technologies can provide answers within hours as opposed to days. One retrospective study found that a faster time to positivity between blood drawn centrally versus peripherally could effectively determine if the septic source was the indwelling catheter or another source.49 Special attention should be given to the patient who presents with severe diarrhea and a history of even a single dose of antibiotics, especially clindamycin or cefoxitin, with a white blood cell count > 30 × 109 per mL. These patients should be empirically treated for C. diff colitis with either oral vancomycin or combination of oral vancomycin and intravenous metronidazole.50
In addition to source control, the initialization of antimicrobial therapy is of utmost importance (Table 12.1). SSC guidelines recommend the start of broad-spectrum antimicrobials happen as soon as possible and within the first hour.32 If difficulty arises in obtaining blood cultures, this should not hinder the start of appropriate antimicrobial coverage. A study by Kumar et al. demonstrated that the duration a patient spends hypotensive prior to initiating broad-spectrum, antimicrobials has a direct effect on mortality. Time is truly of the essence. In their study, starting appropriate antimicrobials within the first hour was associated with a survival rate of 79.9%; the average rate of decline in survival was 7.6% per hour; by the sixth hour, if effective antimicrobials were not initiated, mortality rate was an abysmal 58%.51 Therefore, empiric broad-spectrum antimicrobials (potentially providing double coverage) to cover all possible organisms based on the suspected source of infection must be initiated as soon as possible.
TABLE 12.1
ANTIMICROBIAL THERAPY IN THE TREATMENT OF SEPSIS AND SEPTIC SHOCK
De-escalation of therapy according to organism sensitivities must occur as they become available and should occur within 4–5 d.
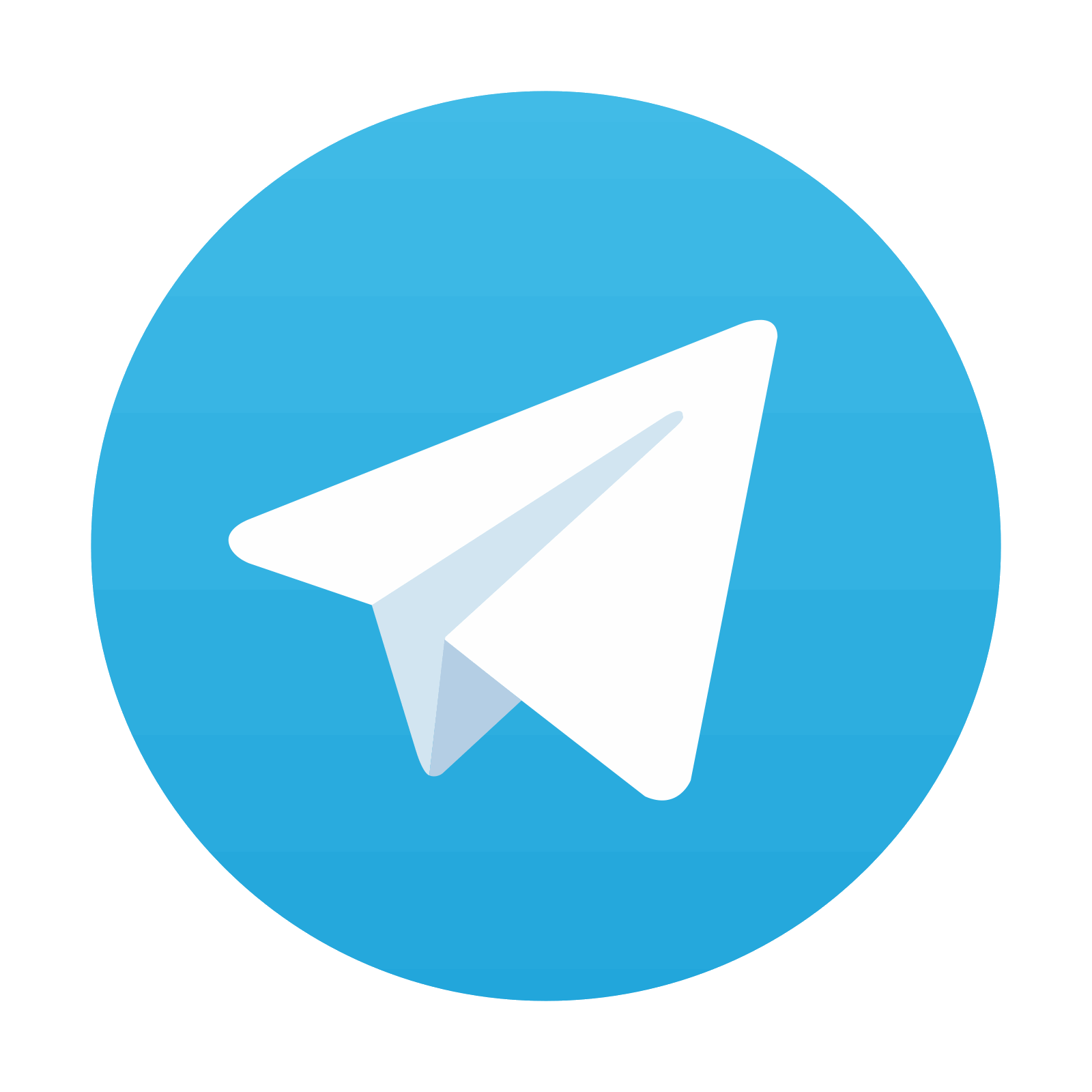
Stay updated, free articles. Join our Telegram channel
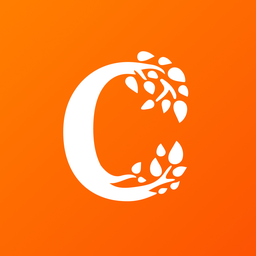
Full access? Get Clinical Tree
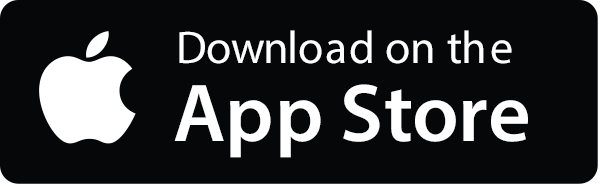
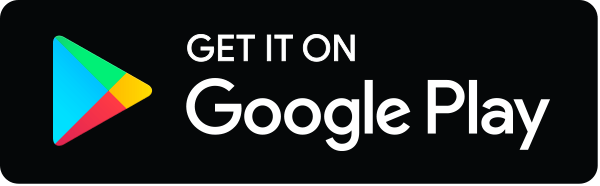