© Springer-Verlag Berlin Heidelberg 2015
Hans-Georg Schaible (ed.)Pain ControlHandbook of Experimental Pharmacology22710.1007/978-3-662-46450-2_8The Role of Glia in the Spinal Cord in Neuropathic and Inflammatory Pain
(1)
Wolfson Centre for Age Related Diseases, King’s College London, London, UK
Abstract
Chronic pain, both inflammatory and neuropathic, is a debilitating condition in which the pain experience persists after the painful stimulus has resolved. The efficacy of current treatment strategies using opioids, NSAIDS and anticonvulsants is limited by the extensive side effects observed in patients, underlining the necessity for novel therapeutic targets. Preclinical models of chronic pain have recently provided evidence for a critical role played by glial cells in the mechanisms underlying the chronicity of pain, both at the site of damage in the periphery and in the dorsal horn of the spinal cord. Here microglia and astrocytes respond to the increased input from the periphery and change morphology, increase in number and release pro-nociceptive mediators such as ATP, cytokines and chemokines. These gliotransmitters can sensitise neurons by activation of their cognate receptors thereby contributing to central sensitization which is fundamental for the generation of allodynia, hyperalgesia and spontaneous pain.
Keywords
GliaMicrogliaAstrocytesNeuropathic painInflammatory painSpinal cordCX3CL1/R1IL-1βTNFRheumatoid arthritis1 Origin and Function of Glia
The term neuroglia was coined to describe the interstitial substance surrounding neurons of the CNS (central nervous system) and was later determined to consist of distinct neuroglial cells. The name astrocyte was introduced to describe ‘star-shaped’ neuroglial cells which were observed to form the supportive system of the CNS. A third cellular element of the CNS was acknowledged in 1913 when improved staining techniques for neuroglial cells led to the recognition of non-neuronal cells that were distinct from astrocytes. During the 1920s del Rio-Hortega employed silver carbon staining and light microscopy to visualise a population of cells that appeared different from the macroglia (oligodendrocytes and astrocytes) that had previously been described in the CNS. These cells, termed microglia, were thought to arise either from CNS invasion of blood mononuclear (monocytic) cells or mesodermal pial elements (Del Rio-Hortega 1932, 2012a, b; Kettenmann et al. 2011; Prinz and Mildner 2011). Whilst alternatives for the origin of microglia have been hypothesised, evidence to support the hypothesis that these cells are of monocytic lineage was later strengthened using autoradiography. Leblond and colleagues demonstrated that microglia possess specific monocytic characteristics, specifically the ability to transform from an amoeboid to a ramified cell (Imamoto and Leblond 1978; Ling et al. 1980). The monocytic/myeloid origins of microglia has now been confirmed conclusively by the absence of microglia from the CNS of a genetically altered mouse strain that lack PU.1, a key transcription factor in the control of myeloid cell differentiation (McKercher et al. 1996; Beers et al. 2006).
Microglia appear at an early stage during embryogenesis where they originate from macrophages in the foetal yolk sac that migrate into the CNS (Saijo and Glass 2011); evidence from postnatal fluorescently labelled cells demonstrates that in this phase of development, microglia are capable of differentiating from monocytes entering the CNS from the circulation (Perry et al. 1985). Conversely, in adult rodents, circulating monocytes only enter the CNS under conditions where there is disruption to the blood-brain barrier (BBB) (King et al. 2009); however, as demonstrated by the use of immune-irradiated mice, the CNS colonisation by these cells is transient and does not contribute to the resident microglial cell pool (Ajami et al. 2011). Rather the microglial population is maintained locally and independently of circulating monocytes via the proliferation of existing cells (Lawson et al. 1992; Ginhoux et al. 2010).
Microglia are specialised phagocytes of the CNS and constitute 5–20 % of the total glial cell population (Saijo and Glass 2011). Under physiological conditions microglia exist in a ‘resting’ or ‘quiescent’ state; these cells can be distinguished morphologically by their small soma and ramified process that perform immune surveillance of the surrounding area. Additionally they express receptors for complement components (Fcγ receptor of IgG) and exhibit low expression of cell surface antigens (Nimmerjahn et al. 2005). Several mechanisms by which these cells maintain a quiescent state have been proposed, including interaction of microglial CX3CR1 receptor with its neuronal ligand the chemokine CX3CL1 and inhibitory signalling through the microglial cell surface proteins CD172, CD200R and CD45 with their neuronal ligands CD47, CD200 and CD22, respectively (Ransohoff and Perry 2009; Ransohoff and Cardona 2010; Cardona et al. 2006; Saijo and Glass 2011).
Within the healthy CNS, microglia have a number of key roles in addition to their function as immune surveyors. As well as monitoring extrasynaptic regions, microglial processes transiently contact synapses, including presynaptic terminals and perisynaptic clefts (Tremblay et al. 2010). Additionally these cells possess a number of neurotransmitter receptors; combined these attributes allow microglia to monitor synaptic function (Salter and Beggs 2014). Microglial cells are present in the CNS from the early stages of development and due to their phagocytic capacity are able to contribute to the elimination of excess neurons that form as part of normal development (Marin-Teva et al. 2011). However, rather than simply removing waste, microglia can initiate apoptosis of cells via the release of several factors including superoxide ions and TNF (Marin-Teva et al. 2004; Sedel et al. 2004; Salter and Beggs 2014). Additionally, a number of inappropriate synaptic connections are made between neurons during development, and microglia play an important role in the regulation of these contacts via the process of synaptic pruning—the phagocytosis of both pre- and postsynaptic elements in a complement cascade-dependent manner (Salter and Beggs 2014; Stevens et al. 2007; Schafer et al. 2012). Microglia contribute to the maturation of established synapses; the functional properties of synapses develop abnormally in mice deficient in several microglial proteins, including CX3CR1, for example, the absence of which results in increased excitatory neurotransmission (Paolicelli et al. 2011; Salter and Beggs 2014). The involvement of microglia to the maintenance of synaptic plasticity has also been investigated in the adult CNS, where they contribute to both homeostatic and activity-triggered plasticity by releasing a number of mediators such as TNF and TGFβ (Butovsky et al. 2014; Koeglsperger et al. 2013).
Of all of the glial cell populations within the CNS, astrocytes are by far the most abundant. In mammals astrocytogenesis begins in late embryogenesis and continues into the postnatal phase. The origin of astrocytes is likely diverse, varying throughout the stages of development. For example, within the cerebral cortex, astrocytes develop from two distinct sources: from radial glia in the ventricular zone during embryogenesis and from subventricular zone (SVZ) progenitors during the postnatal period (Wang and Bordey 2008). Additionally, studies using in vivo retroviral gene transfer have demonstrated that SVZ progenitors can generate both grey and white matter astrocytes as well as oligodendrocytes (Levison and Goldman 1993, 1997; Levison et al. 1993).
The typical image of an astrocyte is that of a stellate cell; however, astrocytes have a complex and heterogeneous morphology (Wang and Bordey 2008). Whilst the nomenclature is considered outdated by some, astrocytes are typically classified into one of two subtypes, protoplasmic and fibrous, where the former are found throughout the grey matter and possess branches that give rise to uniformly distributed processes and the latter are distributed within the white matter and exhibit long fibrelike processes (Sofroniew and Vinters 2010). Furthermore, the branching processes of protoplasmic astrocytes envelop synapses and have endfeet that encase blood vessels, whilst the endfeet of the unbranched processes of fibrous astrocytes envelop nodes of Ranvier (Wang and Bordey 2008). Immunohistochemically astrocytes are commonly identified by the presence of glial fibrils, the major component of which is glial fibrillary acidic protein (GFAP). Antibodies against S100B, a member of the S100 family of EF-band calcium binding proteins, are also used to identify astrocytes; however, this antigen is only expressed by a subset of mature astrocytes. Thus, its expression is not representative of the astrocyte population as a whole (Baudier et al. 1986; Deloulme et al. 2004; Hachem et al. 2005).
Due to the absence of several biophysical properties such as the ability to generate action potentials under physiological conditions, astrocytes were once described as passive (Steinhauser et al. 1992); however, this term is now rarely used. Whilst the initial function of astrocytes was understood to be as little more than an inert scaffold to neurons, it is now widely accepted that they play a critical role in a vast number of processes within the CNS (Volterra and Meldolesi 2005). The close association of astrocytes with many neuronal elements allows astrocytes to regulate the extraneuronal environment around synapses and provide support and nourishment to these cells (Gao and Ji 2010), for example, by buffering extracellular potassium and glutamate. Additionally astrocytes have been demonstrated to significantly contribute to neuronal survival and maturation (via the synthesis and release of growth and trophic factors such as NGF), synapse formation and the regulation of angiogenesis and are a major source of adhesion and extracellular matrix proteins within the CNS that can both promote and inhibit neurite growth (Wang and Bordey 2008).
2 Acute and Chronic Pain
Pain is a subjective sensory experience associated with actual or potential tissue damage (www.iasp.org). It is always unpleasant and therefore an emotional experience. Acute pain is mediated by multi-synaptic pathways beginning with specialised primary afferent fibres (nociceptors) in the periphery, whose cell bodies lie in the dorsal root ganglia (DRG), via the dorsal horn of the spinal cord to supraspinal sites such as the parabrachial area (PBA), periaqueductal grey (PAG), thalamus and cortex. The nociceptors convert the energy of noxious stimuli into electrical impulses which are transmitted to the dorsal horn of the spinal cord. Within the spinal cord, nociceptive transmission is mediated predominately by glutamate acting upon postsynaptic ionotropic receptors; however, the co-release of substance P and calcitonin gene-related peptide (CGRP) and the subsequent activation of their respective NK1 and CGRP receptors postsynaptically modulates glutamatergic transmission (Go and Yaksh 1987; Cheunsuang and Morris 2000; Malcangio and Bowery 1994; Lever et al. 2003; Oku et al. 1987; Seybold et al. 2003). Modulatory control of acute pain is provided by descending inhibitory and facilitatory pathways from the brain and from inhibitory and excitatory interneurons within the spinal cord (Todd 2010; D’Mello and Dickenson 2008).
Acute pain is a vital protective mechanism that persists only for the duration of tissue damage or the presence of a noxious stimulus. Evidence for the importance of acute pain is provided by individuals expressing the rare phenotype of insensitivity to pain; these patients experience regular inadvertent self-mutilation through injury and have a significantly lower than average life expectancy (Cox et al. 2006; Verpoorten et al. 2006).
Commonly pain outlives its usefulness and becomes chronic pain, which is profoundly different from acute pain, as it is the result of plastic changes within the pain pathways. Chronic pain is a debilitating condition lasting longer than 3 months from the noxious stimuli and causes pain to be perceived as out of proportion to the initial inciting injury. Chronic pain can be classified into different types depending on its cause: inflammatory pain is commonly due to tissue inflammation as observed in arthritis, and neuropathic pain arises upon injury to the nervous system.
2.1 Inflammatory and Neuropathic Pain
In both cases a combination of mechanisms results in augmented nociceptive transmission due to peripheral and central sensitisation. Neuropathic pain is that arising from a lesion to the PNS (peripheral nervous system) or CNS as a consequence of physical trauma or disease pathogenesis. Chronic inflammatory pain is typically associated with inflammatory diseases such as arthritis, where the presence of algogenic mediators results in nociceptor sensitisation in the affected tissue, such as the joints in the case of arthritis. Chronic pain is characterised by the presence of a range of symptoms including hyperalgesia (increased sensitivity to noxious stimuli), allodnyia (a painful response to a previously innocuous stimuli) and spontaneous pain (Woolf and Mannion 1999). The treatment of chronic pain is a substantial problem within the clinic as many patients do not respond adequately to available analgesics. The difficulty in treating chronic pain conditions is thought to be a consequence of the heterogeneity of the molecular mechanisms underlying the development and maintenance of chronic pain, many of which are not well understood.
The mechanisms by which these maladaptive pain states arise can be categorised into peripheral sensitisation (changes in peripheral nerves) and central sensitisation (including immune responses in the spinal cord). Peripheral sensitisation due to injury to a localised area leads to primary hyperalgesia and results in increased nociceptive transmission from the periphery which causes changes in dorsal horn neuron activation. Dorsal horn neurons exhibit reduced thresholds to noxious stimuli applied to the periphery, de novo excitation by previously innocuous stimuli, expansion of their receptive fields and increased spontaneous activity. These changes increase excitability of CNS neurons and result in central sensitisation, which is fundamental for the generation of allodynia, secondary hyperalgesia and spontaneous pain (Kuner 2010; Latremoliere and Woolf 2009; Sandkuhler 2009). The resulting increase in glutamate released from central terminals of sensitised neurons trigger phosphorylation of NMDA receptors and post-translational modifications of neuronal proteins. Glial cells within the spinal cord respond to the enhanced nociceptive input by proliferating and switching to a responsive state whereby they release mediators which contribute to dorsal horn mechanisms of chronic pain (Clark and Malcangio 2012; Old and Malcangio 2012). Enhanced activity within the spinal cord triggers cortical and subcortical structures to facilitate excitation and signalling and constitutes higher centre modulation (D’Mello and Dickenson 2008).
Here we will describe and define some of the key mechanisms by which microglia and astrocyte responses in the dorsal horn contribute to the development and maintenance of chronic neuropathic and inflammatory pain states, with a specific focus on chemokine/cytokine signalling and second messenger activation.
3 Spinal Glia Changes in Models of Neuropathic Pain
3.1 Microglial Responses to Injury or Insult
Microglia are the resident immune cells of the CNS and as such respond to pathological insult or tissue injury and subsequent release of mediators from damaged cells. These cells respond to a number of mediators that are increased in the dorsal horn following peripheral injury. They express receptors for the neurotransmitters released from the central terminals of primary afferents: glutamate NMDA and AMPA receptors, the SP receptor NK1 and the CGRP receptor, TrkB receptors for brain derived neurotrophic factor (BDNF) and purinergic receptors including P2X7 and P2X4, many of which are upregulated following injury to the periphery (Rasley et al. 2002; Pezet et al. 2002; McMahon and Malcangio 2009; Ransohoff and Perry 2009).
Microglia respond quickly to an insult by proliferating to expand their population; this process is often termed microgliosis. An insult need not be central in nature, for example it could be the result of a CNS infection or spinal cord injury. In rodent peripheral nerve injury models of neuropathic pain, microglia within the dorsal horn of the spinal cord respond swiftly to augmented primary afferent fibre input. This phenotypic shift is often referred to as the ‘activation’ of microglia. Input from the periphery is vital for this process; blockade of peripheral nerve conduction by the application of a local anaesthetic prevents nerve injury-induced microglial response (Wen et al. 2007; Hathway et al. 2009; Suter et al. 2009). In the region of the dorsal horn in which the injured primary afferents terminate, microglia increase in their numbers and appear more amoeboid, with a hypertrophied soma and thick retracted processes. Additionally they alter their expression of cell surface antigens that play a critical role in immune responses; for example, MHC class II is increased allowing the presentation of antigens by microglia (McMahon and Malcangio 2009). Activated microglia also synthesise and release a variety of pro-nociceptive mediators into the extracellular environment, including cytokines (e.g. TNF and IL-1β), chemokines (e.g. CCL2), reactive oxygen species (ROS) and nitric oxide (NO). Prominent signalling pathways in the development of neuropathic pain are the CX3CR1/L1 loop (discussed below) and the purinergic pathway of microglial activation and subsequent BDNF release. ATP is known to stimulate microglia both in vivo and in vitro via purinergic receptors on the surface membrane of the cells (Honda et al. 2001; Tsuda et al. 2003, 2012; Davalos et al. 2005). Microglial P2X4 is of particular importance for the development of neuropathic pain; it is upregulated in microglia as early as 24 hours after peripheral nerve injury, and the pharmacological inhibition of this receptor transiently attenuates mechanical allodynia in rodents. Furthermore, the intrathecal administration of ATP-treated microglia in naive mice results in the development of mechanical hypersensitivity (Tsuda et al. 2003; Coull et al. 2005). Current evidence indicated that downstream effects of the activation of microglial purinergic receptors are mediated by the release of BDNF via the phosphorylation of p38 MAPK; the administration of an inhibitor of the BDNF receptor, TrkB, prevents the behavioural changes observed following the administration of activated microglia (Coull et al. 2005), and a p38 MAPK inhibitor is able to prevent release of BDNF from microglia (Trang et al. 2009).
The temporal profile of microglial activation within the spinal cord is well documented in models of neuropathic pain. Real-time PCR of spinal cord tissue extracts demonstrates alterations in the levels of microglia-specific mRNA transcripts (e.g. TLR4 and CD14) within hours of injury (Tanga et al. 2004), and the phosphorylation of the microglial MAPK p38 is evident within minutes from peripheral nerve injury (Svensson et al. 2005b; Clark et al. 2007b). Morphological changes (the presence of amoeboid cells and increased immunoreactivity for the microglial marker Ox42) have been observed immunohistochemically as early as 3 days after nerve injury and are maintained for at least 50 days post-surgery (Clark et al. 2007a). Furthermore, the temporal profile of microglial activation is concomitant to the development of behavioural hypersensitivity (Clark et al. 2007b; Zhang et al. 2007; Peters et al. 2007) and the intrathecal administration of compounds that inhibit glial activation to neuropathic rodents is able to attenuate pain behaviours (Clark et al. 2007a; Tawfik et al. 2007), demonstrating that the activation of these cells does indeed contribute to aberrant pain signalling.
3.2 Astrocytic Responses to Injury or Insult
Due to their many functions in the maintenance of CNS homeostasis, under physiological conditions astrocytes are often referred to as ‘active’. Following a change in their environment, such as that occurring in the spinal cord in models of chronic pain, these cells shift to a ‘reactive’ phenotype; astrocytes undergo hypertrophy and increase their expression of a number of cellular proteins including GFAP, S100β and vimentin, which are consequently often used as markers of astrocyte reactivity (Ridet et al. 1997; Pekny and Nilsson 2005). This process is referred to as astrogliosis and has been demonstrated in a number of surgical models of neuropathic pain (Garrison et al. 1991; Colburn et al. 1999; Sweitzer et al. 1999). Astrocyte reactivity can occur through the activation of several pathways; these cells express the receptors for the neurotransmitters NMDA, SP and CGRP (Porter and McCarthy 1997) and thus respond to increased transmitter release in the dorsal horn of the spinal cord subsequent to peripheral nerve injury. In addition, astrocytes possess a variety of cytokine and chemokine receptors that can be activated by their ligands released from microglia; key examples include IL-18/IL-18R, TNF/TNFR and CCL2/CCR2 (Miyoshi et al. 2008; Gao et al. 2009, 2010b). One of the astrocytic changes most likely to be responsible for the contribution of these cells to the maintenance of pain is a decrease in the glutamate transporters GLT1 and GLAST. These transporters function to regulate extracellular glutamate concentrations at non-toxic levels. A decrease in their expression or function results in an elevation in the concentration of spinal extracellular glutamate which can elicit nociceptive hypersensitivity via the activation of NMDA and AMPA receptors (Liaw et al. 2005; Weng et al. 2006). Recent evidence indicates that astrocytes, like microglia, play a critical role in aberrant pain signalling, as the administration of glial inhibitors, such as fluorocitrate, attenuates pain behaviours in rodents (Milligan et al. 2003; Watkins et al. 1997; Okada-Ogawa et al. 2009). Interestingly, the intrathecal administration of reactive astrocytes, briefly incubated with TNF, induces the development of mechanical allodynia in uninjured mice (Gao et al. 2010b). As with microglia, the temporal profile of astrocyte activation has been assessed; astrogliosis becomes apparent after the microglial response, several days after peripheral nerve injury, and lasts for over 4 weeks post-injury (Colburn et al. 1999; Tanga et al. 2004; Romero-Sandoval et al. 2008). Additionally genetically altered mice that are deficient in GFAP develop a shorter lasting mechanical allodynia than their wild-type counterparts. Finally the administration of a GFAP antisense mRNA that prevents translation of GFAP reverses established mechanical allodynia when administered to rats 6 weeks following a peripheral nerve injury (Kim et al. 2009). Together these data demonstrate a role for astrocytes in the maintenance of neuropathic pain.
3.3 CX3CL1, CX3CR1 and Cathepsin S
CX3CL1, also known as fractalkine, is the only member of the CX3C family of cytokines. The cx3cl1 gene was originally described as abundant in the brain and heart but present in all tissues assessed, except peripheral blood leukocytes (Pan et al. 1997). Recent evidence obtained from a series of in situ hybridisation and immunohistochemical studies has determined that neurons are the principle source of CX3CL1 in the CNS (Hughes et al. 2002; Nishiyori et al. 1998; Tarozzo et al. 2003). Additionally, the development of a transgenic mouse expressing a red fluorescent reporter under the control of the CX3CL1 promoter has demonstrated that within the spinal cord CX3CL1 is expressed in dorsal horn neurons (Kim et al. 2011). Both the CX3CL1 protein and mRNA are expressed here, but the protein is not upregulated following peripheral nerve injury (Verge et al. 2004; Clark et al. 2009; Lindia et al. 2005). Furthermore, it has been demonstrated that cytoplasmic glutamate-containing vesicles are present within CX3CL1-positive neurons, indicating that CX3CL1 is expressed within excitatory neurons (Tong et al. 2000).
First described as a potent chemoattractant of T-cells and monocytes in the late 1990s (Bazan et al. 1997; Pan et al. 1997), CX3CL1 exists in two forms. As a membrane-tethered protein it plays a critical role in the firm adhesion of leukocytes to the endothelium to facilitate transmigration (Imai et al. 1997; Fong et al. 1998; Corcione et al. 2009). Soluble CX3CL1 is produced by metalloproteases (ADAM10/17) or protease (Cathepsin S) -mediated cleavage of membrane-bound CX3CL1. Whilst ADAM 10 is responsible for constitutive shedding of CX3CL1, ADAM 17 facilitates inducible shedding of this protein (Bazan et al. 1997; Hundhausen et al. 2003, 2007; Clark et al. 2011). Cathepsin S, on the other hand, is expressed by antigen-presenting cells such as microglia where its release is dependent on the activation of the purinergic receptor P2X7 (Clark et al. 2010) and is enhanced by pro-inflammatory mediators (Liuzzo et al. 1999a, b). CX3CL1 exerts its biological effects by binding to CX3CR1 for which it is the only ligand. Expression analysis of CX3CR1 has demonstrated its mRNA to be abundant in the spleen and peripheral blood leukocytes as well as the brain. Furthermore, the expression of CX3CR1 mRNA is tenfold higher in cultured microglial than whole brain samples, suggesting the receptor is of microglial origin. FACS analysis and calcium imaging of microglia have demonstrated that these cells possess the functional CX3CR1 protein as well as the mRNA (Harrison et al. 1998). This expression profile of CX3CR1 has been confirmed conclusively by the development of a transgenic mouse in which the CX3CR1 contains a green fluorescent protein reporter allowing visualisation of the transcribed protein; here, in the mouse, CX3CR1 protein was observed in microglia throughout the CNS but was not present in astrocytes or oligodendrocytes (Jung et al. 2000).
Within the CNS the CX3CL1–CX3CR1 interaction contributes the maintenance of a quiescent phenotype in microglia and suppresses the release of pro-inflammatory mediators (Mizuno et al. 2003; Lyons et al. 2009). As such, under these conditions, this protein–protein relationship is thought to be neuroprotective. In the context of chronic pain, several observations support a pro-nociceptive role of CX3CL1. The administration of the soluble chemokine domain of CX3CL1 into the intrathecal space at the lumbar level is pro-nociceptive and causes otherwise naive animals to exhibit nocifensive behaviours (Zhuang et al. 2007; Clark et al. 2007b; Milligan et al. 2004, 2005a). Consistently, the intrathecal administration of anti-CX3CL1 antibodies to neuropathic rodents attenuates pain-related behaviour (Clark et al. 2007b). Interestingly, the CSF levels of CX3CL1 increase in neuropathic animals compared to sham controls (Clark et al. 2007b, 2009). CX3CR1 exhibits similar pro-nociceptive attributes under aberrant pain conditions. Enhanced expression of the protein within the dorsal horn of the spinal cord is associated with microgliosis following peripheral nerve injury (Zhuang et al. 2007; Staniland et al. 2010). One mechanism described is IL-6 dependent; IL-6 mRNA and protein expression is induced in neurons following the peripheral nerve injury (Arruda et al. 1998; Lee et al. 2009), and prophylactic treatment with an IL-6 neutralising antibody prevents increased CX3CR1 expression, whilst, conversely, the administration of recombinant IL-6 significantly augments CX3CR1 expression (Lee et al. 2010). Supporting a pro-nociceptive role of CX3CR1 and a critical role for CX3CL1–CX3CR1 interaction in the development of pathological pain responses, CX3CR1-deficient mice do not develop hyperalgesia and/or allodynia in models of nerve injury and exhibit reduced microgliosis when compared to their wild-type littermate controls (Staniland et al. 2010). Similarly, the intrathecal administration of an anti-CX3CR1 antibody attenuates both the behavioural and microglial responses to injury (Zhuang et al. 2007; Milligan et al. 2004, 2005a). Within spinal cord microglia activation of CX3CR1 by CX3CL1 results in increased intracellular calcium concentrations (Harrison et al. 1998), the phosphorylation of p38 MAPK and subsequent release of pro-nociceptive molecules such as IL-6, NO and IL-1β (Zhuang et al. 2007; Clark et al. 2007b).
3.4 TNF and TNFR
TNF, previously known as TNFα, is a small pro-inflammatory cytokine first described in activated macrophages as a molecule with tumour-regression activity (Carswell et al. 1975). TNF belongs to a superfamily of ligand/receptor proteins that share a structural motif — the TNF homology domain. The TNF receptors are the other members of this family of proteins; two have been identified and are either constitutively expressed (TNFR1/p55-R) or inducible (TNFR2/p75-R) (Bodmer et al. 2002; Leung and Cahill 2010). Under physiological conditions TNF is expressed at very low levels in the spinal cord; however, it is rapidly upregulated in both microglia and astrocytes (glia) and neurons following peripheral injury (DeLeo et al. 1997; Ohtori et al. 2004; Hao et al. 2007; Youn et al. 2008). Similarly both TNFR1 and TNFR2 are expressed within glia and neurons in the spinal cord (Gruber-Schoffnegger et al. 2013; Ohtori et al. 2004; Hao et al. 2007). The use of receptor-specific protein ligands has demonstrated that it is TNFR1 activation in the spinal cord that is responsible for the pro-nociceptive effects of TNF under steady-state conditions; TNFR2, on the other hand, contributes to the pro-nociceptive effects of TNF under chronic pain states following its upregulation after nerve injury (Liu et al. 2007; Clark et al. 2013).
In the context of peripheral nerve injury models of neuropathic pain, TNF is detectable in the periphery at the site of injury where it is upregulated and exerts pro-nociceptive functions (George et al. 1999; Shubayev and Myers 2000; Sommer and Schafers 1998; Leung and Cahill 2010). Within the spinal cord TNF exerts its pro-nociceptive effects via actions on both neurons and glia. The spinal administration of TNF is associated with the development of mechanical allodynia and thermal hypersensitivity (Youn et al. 2008; Zhang et al. 2011). Additionally the application of exogenous TNF to spinal cord slices induces rapid modulation of Aδ- and C-fibre (nociceptive-fibre)-mediated neurotransmission (Youn et al. 2008), enhances dorsal horn neuronal responses to C-fibre stimulation (Reeve et al. 2000) and augments excitatory neurotransmission in lamina II neurons in a predominantly TNFR1-dependent manner (Zhang et al. 2011). Additionally long-term potentiation (LTP) induced by stimulation of the sciatic nerve is attenuated in TNFR knock-out mice (Park et al. 2011). These effects may not be mediated directly by the activation of neuronal TNF receptors, but rather indirectly by the activation of receptors located on glial cells within the dorsal horn (Gruber-Schoffnegger et al. 2013). Indeed, TNF is also able to modulate glial cell activation within the spinal cord as blockade of TNF signalling is associated with a reduction in glial cell activity in models of peripheral nerve injury (Svensson et al. 2005b; Nadeau et al. 2011). Via P2X7 signalling ATP is able to induce TNF production in microglia by a P38 MAPK-dependent pathway (Suzuki et al. 2004; Lister et al. 2007); in rats with a peripheral nerve injury, concomitant increases of TNF and p-P38 are observed in the spinal cord, and blockade of TNF suppresses P38 phosphorylation and microgliosis (Schafers et al. 2003; Marchand et al. 2009). Evidence from cultured astrocytes demonstrates that TNF transiently activates JNK (Gao et al. 2009), a mitogen-activated kinase (MAPK) associated with the synthesis and release of pro-nociceptive mediators (Ji et al. 2009). In particular this pathway is associated with the upregulation of astrocytic CCL2; CCL2 upregulation by TNF is dose dependently inhibited by a JNK inhibitor (Gao et al. 2009). Similarly, the spinal injection of TNF produces JNK-dependent behavioural hypersensitivity, whilst the administration of a JNK inhibitor to nerve-injured animals suppresses pain-associated behaviour (Gao et al. 2009). Peripheral nerve injury induces a slow but persistent activation of JNK MAPK particularly within astrocytes in the spinal cord. Whilst the administration of a specific JNK inhibitor has no effect on acute pain responses, it potently prevents and reverses nerve injury-induced mechanical allodynia in rodents (Zhuang et al. 2006).
3.5 IL-1β and IL-1R
Interleukin-1β (IL-1β) is a small pro-inflammatory cytokine first described as a pyrogenic factor released from leukocytes (Dinarello 2007), and was one of the first cytokines to be implicated in the mechanisms underlying enhanced nociception in rodents following peripheral nerve injury (Clark et al. 2013). IL-1β, a 17.5 kDa protein, is part of the well-characterised IL-1 family of proteins that also includes IL-1α, the IL-1 receptor (IL-1R1), a decoy receptor (IL-1R2) and the endogenous receptor antagonist (IL-1ra). IL-1ra is structurally similar to IL-1β and binds to IL-1R1 with similar affinity; however, it lacks the ability to activate the receptor and stimulate downstream signalling. Similarly, IL-1R2 is able to bind IL-1β with comparable affinity to IL-1R1 but lacks the appropriate cytosolic region to activate downstream signalling proteins (Weber et al. 2010; Dunn et al. 2001). Within the CNS IL-1β is expressed at low levels by both neurons and glial cells (Ren and Torres 2009; Clark et al. 2006; Copray et al. 2001; Guo et al. 2007; Sommer and Kress 2004), and the expression of IL-1R1 is equally widespread within the spinal cord (Zhang et al. 2008; Sweitzer et al. 1999; Gruber-Schoffnegger et al. 2013).
Under physiological conditions IL-1β has a number of homeostatic functions such as regulation of sleep and temperature (Dinarello 1996). More importantly, IL-1β is a key regulator of pro-inflammatory responses and the control of innate immune responses to pathogen-associated danger signals [pathogen-associated molecular patterns (PAMPS) and danger-associated molecular patterns (DAMPS)], such as lipopolysaccharide (LPS) and bacterial DNA. The mechanism by which IL-1β is secreted from cells differs from the classical ER–Golgi route of protein secretion due to a lack of a leader sequence (Rubartelli et al. 1990). Rather, IL-1β is synthesised as a larger precursor protein and is cleaved into a mature form; the mechanism behind this has now been elucidated and involves caspase-1, which cleaves IL-1β, and a series of accessory proteins that form a complex known as the inflammasome that provides a platform for the activation of caspase (Martinon et al. 2002; Schroder and Tschopp 2010).
Similarly to TNF, IL-1β is pro-nociceptive when administered centrally; the intrathecal administration of exogenous IL-1β results in thermal and mechanical hypersensitivity (Gruber-Schoffnegger et al. 2013; Reeve et al. 2000; Sung et al. 2004; Kawasaki et al. 2008). Further evidence for a pro-nociceptive effect of IL-1β comes from rodents lacking this protein which exhibit attenuated behavioural responses to peripheral nerve injury (Wolf et al. 2006). Furthermore it has been observed that human patients with a range of painful neuropathies exhibit enhanced CSF levels of IL-1β (Alexander et al. 2005; Backonja et al. 2008). Following peripheral nerve injury, IL-1β is upregulated in the spinal cord where it is expressed primarily by glial cells but also by neurons (Clark et al. 2013). Inhibition of normal IL-1β signalling is anti-nociceptive in animal models of neuropathic pain; intrathecal administration of IL-1ra can both prevent and reverse (Milligan et al. 2005b, 2006) nocifensive behaviours in these animals, as can inhibition of caspase-1 which prevents the proteolytic activation of IL-1β, resulting in decreased secretion of the mature form from microglia (Clark et al. 2006).
IL-1β released from glial cells is able to modulate neuronal activity. This cytokine can facilitate glutamatergic transmission via the phosphorylation of the NMDA receptor (Gruber-Schoffnegger et al. 2013) which enhances behavioural hypersensitivity; treatment with IL-1ra attenuates both the behavioural phenotype and receptor phosphorylation. The mechanism underlying this modulation is complex and calcium dependent (Viviani et al. 2003), and involves several intracellular signalling proteins including PKC, IP3, PLC, PLA2 and src kinases. Inhibitors of these proteins are able to block IL-1β-induced phosphorylation of either the NR1 subunit or NR2A/B subunit of the NMDA receptor in vitro similarly to IL-1ra (Viviani et al. 2003). The application of exogenous IL-1β to spinal cord slices is able to increase AMPA-mediated currents in lamina II neurons (Kawasaki et al. 2008) and induce LTP within lamina I neurons (Gruber-Schoffnegger et al. 2013). Patch clamp studies of IL-1β-treated spinal cord slices demonstrate that IL-1β enhances excitatory neurotransmission whilst attenuating inhibitory neurotransmission (Kawasaki et al. 2008). Overall these data indicate that IL-1β contributes to a potentiation of excitatory transmission and a suppression of inhibitory transmission, which together correlate with the facilitation of behavioural hypersensitivity.
As with many other cytokines, as well as a neuronal effect, IL-1β is thought to contribute to a pro-nociceptive state via the activation of glia. Additionally glia may contribute to the effects of IL-1β on neuronal excitability as these are absent following the inhibition of glial cell function (Gruber-Schoffnegger et al. 2013; Liu et al. 2013).
4 Spinal Glia During Inflammatory Pain
Inflammation is a critical protective mechanism occurring in response to injury, infection or irritation. It is characterised by five defining components: redness, heat, swelling, loss of function and pain. Under physiological conditions inflammation allows removal or repair of damaged tissue following an injury to the organism. Under these circumstances the role of inflammatory pain is protective, limiting the use of the affected area and preventing further damage during the healing process. However, in patients with chronic inflammatory conditions, such as arthritis (see section below), chronic pain hypersensitivity is a common complaint (Walsh and McWilliams 2014). The continued presence of algogenic mediators results in the sensitisation of the peripheral tissues (Schaible et al. 2009). Commonly models which involve the direct administration of an exogenous algogenic substance into the hind-paw of the rodent have been utilised in order to investigate how spinal glial mechanisms contribute to inflammatory pain. However, increasing spinal glial mechanisms are being studied in more clinically relevant models of inflammatory pain, such as arthritis.
An extensive body of evidence supports a role for spinal microglia and astrocytes (glia) in inflammatory pain mechanisms. Peripheral inflammation results in sensitization of spinal neurons (Vazquez et al. 2012; Konig et al. 2014) and glial cell reactivity within the dorsal horn of the spinal cord. Spinal astrogliosis and/or microgliosis have been reported in many inflammatory pain models (Watkins et al. 1997; Sweitzer et al. 1999; Clark et al. 2007a; Raghavendra et al. 2004). Critically, inhibition of glial cell activity during peripheral inflammation reduces pain behaviours substantially (Meller et al. 1994; Watkins et al. 1997; Clark et al. 2007a), suggesting that the activity of these cells in the spinal cord is vital for the full development of inflammatory pain.
A number of the spinal glia mechanisms that have to identified to contribute to neuropathic pain may also play a role in pain that occurs as result of peripheral inflammation. The cytokine/chemokine glial mechanisms described in relation to neuropathic pain above also play a role in inflammatory pain. One such astrocytic mechanism is inflammation-induced phosphorylation of JNK in spinal astrocytes (Gao et al. 2010a). Intraplantar injection of CFA (Gao et al. 2010a) or carrageenan (Bas et al. 2015) induces JNK phosphorylation, and JNK inhibition attenuates inflammatory pain behaviours (Gao et al. 2010a; Bas et al. 2015). This inflammation-induced JNK phosphorylation is regulated by spinal TNF secretion (Bas et al. 2015) as is the case during neuropathic pain (Gao et al. 2010a). One spinal microglial mechanism that regulates inflammatory pain is the phosphorylation of p38 MAPK. Peripheral inflammation induces extensive p38 phosphorylation which is restricted to microglial cells (Svensson et al. 2003a, 2005a). Inhibition of p38 in inflammatory pain models results in attenuation of pain behaviours (Svensson et al. 2003b, 2005a). The activation of many microglial receptors results in intracellular p38 phosphorylation, suggesting that this is a key intracellular signalling pathway during both inflammatory and neuropathic pain. Indeed, it has previously been demonstrated that the microglial CX3CR1 receptor leads to p38 phosphorylation (Clark et al. 2007b) and is critical for the full expression of inflammatory pain (Staniland et al. 2010), as well as neuropathic pain (see above section).
5 Spinal Glia During Rheumatoid Arthritis Pain
Rheumatoid arthritis (RA) is a chronic autoimmune disease characterised by synovial inflammation and joint destruction. The disease aetiology remains unclear but is thought to comprise a complex interplay between environmental and genetic factors. The clinical signs of RA are accompanied by chronic pain, representing a major unmet clinical need. Despite the availability of disease-modifying agents that reduce the clinical signs of RA, the treatment of chronic pain remains inadequate at present (Kidd et al. 2007; Sokka et al. 2007; Dray 2008; Walsh and McWilliams 2014). The mechanisms of RA pathology have been studied extensively using rodent models. Although pain is common in RA patients, it is only relatively recently that pain has been studied in rodent models that replicate the complex mechanisms of this inflammatory disorder. Whilst inflammatory pain states associated with models of mono-arthritis have been extensively characterised, pain that occurs as a result of more clinically relevant poly-arthritic models has only recently been examined.
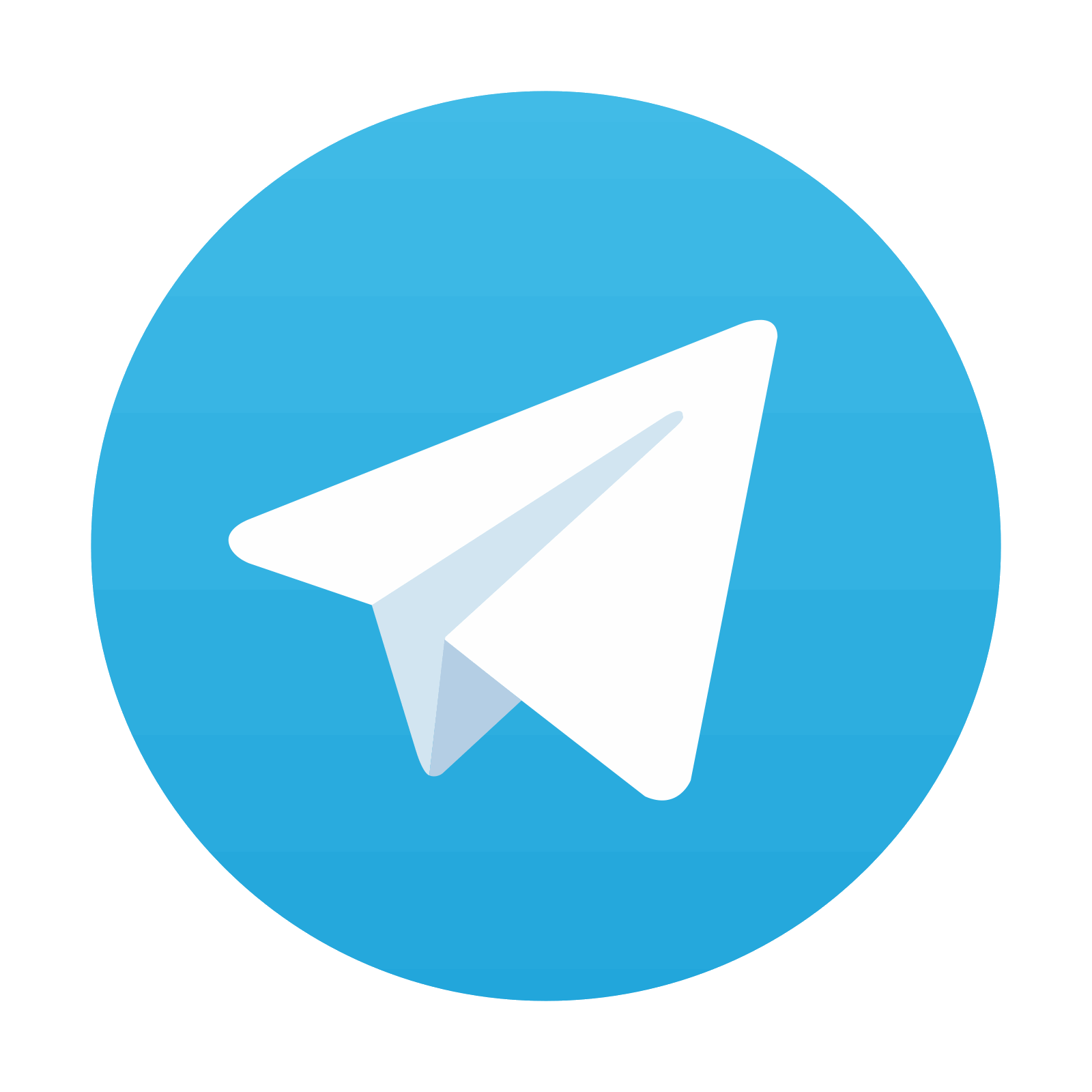
Stay updated, free articles. Join our Telegram channel
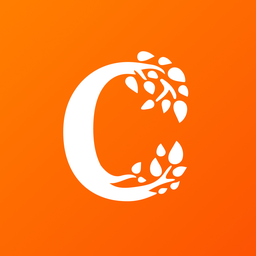
Full access? Get Clinical Tree
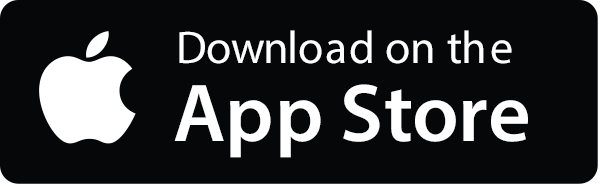
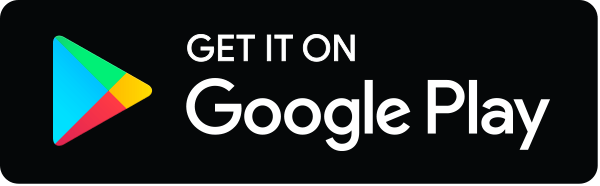