The primary function of the lungs is gas exchange. Specifically, this involves the transport of oxygen (O2) into the blood, and the elimination of carbon dioxide (CO2) from the blood. In addition, the lungs serve as minor organs of metabolism and elimination for a number of xenobiotics, a source of insensible water loss, and a means of temperature regulation. Air is conducted through proximal airways, made up of columnar epithelium and mucin-secreting goblet cells. Oxygen exchange occurs in distal airways, through type I pneumocytes, which make up most of alveolar surface area. Type II pneumocytes, which secrete surfactant, take up less pulmonary surface area.
Cellular oxygen use is dependent on many factors, including respiratory drive; percentage of oxygen in inspired air; airway patency; chest wall and pulmonary compliance; diffusing capacity; ventilation–perfusion mismatch; hemoglobin content; hemoglobin oxygen loading and unloading; cellular oxygen uptake; and cardiac output. Xenobiotics have the unique ability to impair each of these factors necessary for oxygen use and result in respiratory dysfunction (Table 28–1). This chapter illustrates how xenobiotics interact with the mechanisms of gas exchange and oxygen use. Discussion of chronic occupational lung injury is beyond the scope of this text; the reader is referred to a number of reviews for further information.5
Location | Mechanism of Effect | Common Xenobiotics |
---|---|---|
Oxygen displacement | Simple asphyxiation | Carbon dioxide |
Respiratory center | Direct stimulation Inhibition | Methylxanthines Sedatives |
Indirect stimulation | Salicylates | |
Chest wall | Muscular weakness | Botulinum toxin |
Muscular rigidity | Fentanyl | |
Oropharynx | Angioedema Obstruction | ACE inhibitor angioedema |
Trachea | Barotrauma | Nitrous oxide (N2O) |
Tissue destruction | Caustics | |
Larynx | Laryngospasm | Caustics |
Bronchioles | Acute bronchospasm | Chemical irritant |
Delayed bronchospasm | Allergens | |
Alveoli/interstitial | Alveolar permeability (ARDS) | Smoke inhalation |
Alveolar hemorrhage | Bleomycin | |
Diffuse airspace filling | Nitrogen dioxide (NO2) | |
Direct tissue destruction (primary or secondary) | Phosgene | |
Fat embolism syndrome | Intravenous lipid emulsion | |
Multifocal airspace filling | Intravenous drug use (septic emboli) | |
Hypersensitivity pneumonitis | Actinomycetes spp | |
Lymphocytic infiltrates or granulomas | Immune checkpoint inhibitors | |
Metal pneumonitis | ZnCl2 fume | |
Pulmonary surfactant disruption | Hydrocarbons | |
Pulmonary fibrosis | Paraquat (late) | |
Pulmonary vessels | Vasculitis | Levamisole |
Pleura | Pleural plaque | Asbestos |
Hilar lymph nodes | Calcification | Silica |
Lymphadenopathy | Phenytoin |
Respiratory rate and depth are regulated by the need to maintain a normal partial pressure of carbon dioxide (PCO2) and pH. Most of the control for ventilation occurs at the level of the medulla, although it is modulated both by involuntary input from the pons and voluntary input from the higher cortices. Changes in PCO2 are measured primarily by a central chemoreceptor, which measures cerebralspinal fluid pH, and secondarily by peripheral chemoreceptors in the carotid and aortic bodies, which measure PCO2. Input with regard to partial pressure of oxygen (PO2) is obtained from carotid and aortic chemoreceptors. Stretch receptors in the chest relay information about pulmonary dynamics, such as the volume and pressure.
Xenobiotics affect respiratory drive in one of several ways: direct suppression of the respiratory center; alteration in the response of chemoreceptors to changes in PCO2; direct stimulation of the respiratory center; increase in metabolic demands such as those resulting from agitation or fever, which, in turn, increases total body oxygen consumption; or indirectly, as a result of the creation of acid–base disorders. For example, opioids (Chap. 36) depress respiration by decreasing the responsiveness of chemoreceptors to CO2 and by direct suppression of the pontine and medullary respiratory centers.36,87,113 Any xenobiotic that causes a decreased respiratory drive or a decreased level of consciousness can produce bradypnea (a decreased respiratory rate), hypopnea (a decreased tidal volume), or both, resulting in absolute or relative hypoventilation (Chap. 3).
Methylxanthines and sympathomimetics increase both respiratory drive and oxygen consumption. Salicylates produce hyperventilation by both central and peripheral effects via respiratory alkalosis and metabolic acidosis. The net consequence of increased respiratory drive, increased oxygen consumption, or metabolic acidosis is the generation of tachypnea (an elevated respiratory rate), hyperpnea (an increased tidal volume), or both. Either alone or in combination, tachypnea and hyperpnea produce hyperventilation. Tables 28–2 and 28–3 both list xenobiotics that commonly produce hypoventilation and hyperventilation.
Baclofen |
Barbiturates |
Botulinum toxin |
Carbamates |
Clonidine |
Colchicine |
Conium maculatum (poison hemlock) |
Cyclic antidepressants |
Elapid venom |
Ethanol |
Ethylene glycol |
γ-Hydroxybutyrate and analogs |
Isopropanol |
Methanol |
Neuromuscular blockers |
Nicotine |
Opioids |
Organic phosphorus compounds |
Sedative–hypnotics |
Strychnine |
Tetanus toxin |
Tetrodotoxin |
Xenobiotic induced electrolyte abnormalities |
Amphetamines |
Anticholinergics |
Camphor |
Carbon monoxide |
Cocaine |
Cyanide |
Dinitrophenol |
Ethanol (ketoacidosis) |
Ethylene glycol |
Gyromitra spp (Mushroom) |
Hydrogen sulfide |
Iron |
Isoniazid |
Isopropanol |
Methanol |
Metformin |
Methemoglobin inducers |
Methylxanthines |
Nucleoside reverse transcriptase inhibitors |
Paraldehyde |
Pentachlorophenol |
Phenformin |
Progesterone |
Salicylates |
Sodium monofluoroacetate |
Barometric pressure at sea level ranges near 760 mm Hg. At this pressure, 21% of ambient air is composed of oxygen (the fraction of inspired oxygen {FiO2} = 21%), and, after subtracting for the water vapor normally present in the lungs, the alveolar partial pressure of oxygen (PAO2) is about 150 mm Hg. Any reduction in FiO2 decreases the PAO2, thereby producing signs and symptoms of hypoxemia (a low arterial partial pressure of oxygen {PaO2}). At an FiO2 of 12% to 16%, patients experience tachypnea, tachycardia, headache, mild confusion, and impaired coordination. A further decrease to an FiO2 of 10% to 14% produces severe fatigue and cognitive impairment and when the FiO2 decreases to between 6% and 10%, nausea, vomiting, and lethargy develop. An FiO2 less than 6% is incompatible with life.66
This effect on PAO2 is typically observed as elevation increases above sea level, because while FiO2 remains 21%, barometric pressure falls. At 18,000 feet, barometric pressure is only 380 mm Hg, and the PAO2 falls to below 70 mm Hg. At 63,000 feet, the barometric pressure falls to 47 mm Hg, a level where the PAO2 equals 0 mm Hg. Although it is important to remember this relationship, altitude-induced decreases in PAO2 are rarely important in clinical medicine, even in commercial airline flights, in which the cabins are pressurized to a maximum of several thousand feet above sea level. However, in closed or low-lying spaces, oxygen is replaced or displaced by other gases that have no intrinsic toxicity. Common examples of these gases, referred to as simple asphyxiants (Table 28–4), are found alone or in combination with more toxic gases. Because they have little or no toxicity other than their ability to replace oxygen, removal of the victim from exposure and administration of supplemental oxygen are curative if permanent injury as a consequence of hypoxia has not already developed (Chap. 121).
The potential magnitude of toxicity from simple asphyxiants is best exemplified by disasters in Cameroon near Lake Mounoun and Lake Nyos, in 1984 and 1986, respectively. Following an earthquake, Lake Nyos, a volcanic lake, released a cloud of CO2 gas of approximately one-quarter million tons. Because CO2 is 1.5 times heavier than air, the gas cloud flowed into the surrounding low-lying valleys, killing by asphyxia more than 1700 people, and affecting countless more people because of hypoxia. Most survivors recovered without complications.11,40 Smaller-scale, but equally serious, toxicity from CO2 results from improper handling of dry ice or release into a closed space.42,47
Hypoventilation can result from a decrease in either respiratory rate or tidal volume. Thus, even when the stimulus to breathe is normal, adequate ventilation is dependent on the coordination and function of the muscles of the diaphragm and chest wall. Changes in this function can result in hypoventilation by 2 separate mechanisms; both muscle weakness and muscle rigidity impair the patient’s ability to expand the chest wall. Some examples of toxicologic causes of muscle weakness include botulinum toxin,97 electrolyte abnormalities, such as hypokalemia,59,114 or hypermagnesemia,2,33 organic phosphorus compounds,77,98 and neuromuscular blockers.15,52 Patients with hypoventilation caused by muscle weakness respond well to assisted ventilation and correction of the underlying problem (Chaps. 12, 38, 66, and 111). Chest-wall rigidity impairing ventilation can occur in strychnine poisoning,17,57,64 tetanus,24,57,103 and fentanyl use23,25 (Chaps. 36 and 114). Often these patients are difficult to ventilate despite endotracheal intubation and therefore require muscle relaxants, neuromuscular blockers, or naloxone (for fentanyl).
The airway can be compromised in several ways. As a patient’s mental status becomes impaired, the airway is often obstructed by the tongue.44 Alternatively, the presence of vomitus, or aspiration of activated charcoal or a foreign body, directly obstructs the trachea or major bronchi with resultant hypoxia.54,72,90 Obstruction also results from increased secretions produced during organic phosphorus compound poisoning. Laryngospasm occurs either as a manifestation of systemic reactions, such as anaphylaxis, as a result of edema from thermal or caustic injury (Chaps. 103 and 120), or as a direct response to an irritant gas (Chap. 121). Similarly, if the tongue becomes swollen in response to thermal or caustic injury or toxic exposure to plants such as Dieffenbachia spp, or as a result of angioedema from drugs such as angiotensin-converting enzyme inhibitors, airway obstruction results (Chaps. 61, 103, 118, and 120).36 Regardless of the mechanism, upper airway obstruction produces hypoventilation, hypoxemia, and hypercapnia (hypercarbia) with the persistence of a normal alveolar–arterial (A–a) gradient (see discussion of A–a gradients). Upper airway obstruction is often acute and severe and requires immediate therapy to prevent further clinical compromise. Bronchospasm, a common manifestation of anaphylaxis, also results from exposure to pyrolyzed cocaine,96 smoke, irritant gases46 (Table 28–5), dust (eg, cotton in byssinosis), or as a result of work-related asthma63 and hypersensitivity pneumonitis120 (Chaps. 120 and 121).
Pneumothorax is an example of airway collapse caused by barotrauma, which more commonly results from the manner of administration of illicit xenobiotics than from actual drug overdose. Barotrauma also results from nasal insufflation or inhalation of drugs. This form of barotrauma occurs most often in cocaine (particularly in the form of “crack”) and marijuana users, who either smoke or insufflate these xenobiotics and then perform prolonged Valsalva or Mueller maneuvers in an attempt to enhance the effects of these xenobiotics (Chaps. 74 and 75).13,100,116 The increased airway pressure leads to rupture of an alveolar bleb, and free air dissects along the peribronchial paths into the mediastinum and pleural cavities. Nitrous oxide abuse also causes barotrauma.55 Siphoning of nitrous oxide from-low-pressure tanks meant for inhalation is not typically associated with barotrauma. By contrast, inhalation of nitrous oxide, used as a propellant in whipped cream cans, generates tremendous pressure that sometimes results in severe barotrauma (Chaps. 65 and 81).
Ventilation–perfusion (V/Q) mismatch is manifested at the extremes by aeration of the lung without arterial blood supply (as in pulmonary embolism from injected contaminants) and by a normal blood supply to the lung without any ventilation. Impaired blood supply to a normal lung and normal blood supply to an inadequately ventilated lung constitute an infinite number of gradations that exist between the extremes. The normal response to regional variations in ventilation is to shunt blood away from an area of lung poorly ventilated, thereby preferentially delivering blood to an area of the lung where gas exchange is more efficient. A hypoxia-induced reduction in local nitric oxide production appears to be responsible for the regional vasoconstriction that occurs.1 This effect, commonly known as hypoxic pulmonary vasoconstriction, is best described in patients with chronic obstructive lung disease and facilitates compensation for the V/Q mismatch associated with that disorder. It is unclear whether xenobiotic-induced alterations in pulmonary nitric oxide production are significant determinants in the V/Q mismatch that occurs in poisoning.
In toxicology, V/Q mismatch most commonly results from perfusion of an abnormally ventilated lung that results from aspiration of gastric contents, a frequent complication of many types of poisoning.54,78 Although alterations in consciousness and loss of protective airway reflexes are predisposing factors, certain xenobiotics, such as hydrocarbons, directly result in aspiration pneumonitis because of their specific characteristics of high volatility, low viscosity, and low surface tension (Chap. 105).
The diagnosis of aspiration pneumonitis often relies on chest radiography for confirmation. The location of the infiltrate depends on the patient’s position when the aspiration occurred. Most commonly, aspiration occurs in the right mainstem bronchus, because the angle with the carina is not as acute as it is for entry into the left mainstem bronchus. When aspiration occurs in the supine position, the subsequent infiltrate is usually manifest in the posterior segments of the upper lobe and superior segments of the lower lobe. Aspiration typically involves vomitus; however, secretions, activated charcoal, teeth, dentures, food, and other foreign bodies are also frequently aspirated.
Severe impairment in diffusing capacity commonly results from local injury to the lungs in disorders such as interstitial pneumonia, aspiration, toxic inhalations, and near drowning, and from systemic effects of sepsis, trauma, and various other medical disorders.10 When this process is acute and associated with clinical criteria, including crackles, hypoxemia, and bilateral infiltrates on a chest radiograph demonstrating a normal heart size, it has been historically referred to as noncardiogenic pulmonary edema or, acute lung injury (ALI).10 The most severe manifestation of these processes was described as acute respiratory distress syndrome (ARDS). However, a task force of pulmonary specialists determined that all of these conditions are best classified as gradations of ARDS. According to the 2012 Berlin definition, ARDS consists of bilateral pulmonary infiltrates (by radiography or computed tomography), which occur within 7 days of an inciting event and are not explained by heart failure or fluid overload. Severity of ARDS is determined by the PaO2/FiO2 ratio. Acute respiratory distress syndrome is classified as mild (200 mm Hg < PaO2/FiO2 ≤ 300 mm Hg), moderate (100 mm Hg < PaO2/FiO2 ≤ 200 mm Hg), and severe (PaO2/FiO2 ≤ 100 mm Hg). Cases that once were described as ALI now fit under the mild ARDS classification.
Throughout this chapter and text, this newer nomenclature will be used. Using the older definition, it was estimated that 150,000 Americans developed ARDS annually, many as a result of xenobiotics; severe ARDS has a fatality rate approximating 50%.4,35
Commonly, patients are chronically exposed to xenobiotics associated with reduced diffusing capacity by smoking tobacco and other xenobiotics or working with asbestos, silica, and coal, which slowly causes pulmonary fibrosis or promotes emphysema. Chronically smoked cocaine is also recognized to alter pulmonary function.104 Acutely, ARDS from opioids, salicylates, or phosgene and delayed severe fibrosis from paraquat causes profound alterations in diffusion (Chaps. 36, 37, 109, and 126).82,91,121 Associated parenchymal damage is almost always present and causes both reduction in lung volumes and V/Q mismatch. Intravenous injection of talc, a common contaminant found in drugs of abuse,85 and septic emboli from right-sided endocarditis83,102 result in isolated vascular defects with reduction in diffusion capacity. Similarly, cocaine-induced pulmonary artery spasm obstructs vascular channels and alters pulmonary function, creating V/Q mismatch.29
Acute respiratory distress syndrome commonly occurs in cases of poisoning. The edema fluid (and the resulting hypoxia, pulmonary crackles, and radiographic abnormalities) develops in part because of increased permeability of the alveolar and capillary basement membrane.22,26,67,91 Proteinaceous fluid leaks from the capillaries into the alveoli and interstitium of the lung. Several mechanisms are proposed as the cause of ARDS, although no single unifying mechanism exists for all of the xenobiotics implicated. Acute respiratory distress syndrome results from exposure to xenobiotics that produce hypoventilation by at least 3 different mechanisms: (1) hypoxia injures the vascular endothelial cells, (2) autoregulatory vascular redistribution causes localized capillary hypertension, or (3) alveolar microtrauma occurs as alveolar units collapse, only to be reopened suddenly during reventilation.91 These and other events activate neutrophils and release inflammatory cytokines.3,112 Other xenobiotics are directly toxic to the capillary epithelial cells or are at least partly responsible for the release of vasoactive substances.3 The effects of salicylates and other nonsteroidal antiinflammatory drugs are most likely mediated via effects on prostaglandin synthesis. Finally, sympathomimetic stimulants occasionally cause “neurogenic” pulmonary edema, which is likely mediated by massive catecholamine discharge. Elevated catecholamine concentrations are also noted in experimental opioid overdose, possibly representing support for a link between hypoxia, hypercarbia, and the catecholamine hypothesis of ARDS.76
In the 1880s, William Osler described “oedema of left lung” in a morphine user. The opioids are still among the most common causes of ARDS (Chap. 36), but it is now recognized that there are many types of xenobiotics that can cause or are associated with ARDS, such as the sedative–hypnotics, salicylates, cocaine, carbon monoxide, diuretics, and calcium channel blockers (Table 28–6).30,32,34,38,39,45,48,51,58,86,88,101,102,121 The route of xenobiotic administration is not usually the determining factor; ARDS results from oral, intravenous, and inhalational exposures. Because the source of the problem is increased pulmonary capillary permeability, patients with ARDS have a normal pulmonary-capillary wedge pressure, unlike patients with cardiogenic pulmonary edema.
Amiodarone |
Amphetamines |
Amphotericin |
Bleomycin |
Calcium channel blockers |
Carbon monoxide |
Cocaine |
Colchicine |
Cyclic antidepressants |
Cytosine arabinoside |
Ethchlorvynol |
Irritant gases |
Lidocaine |
Opioids |
Protamine |
Salicylates |
Sedative–hypnotics |
Smoke inhalation |
Streptokinase |
Vinca alkaloids |
True cardiogenic pulmonary edema also occurs as the result of poisoning. Etiologies for this phenomenon include the ingestion of xenobiotics with negative inotropy (eg, β-adrenergic antagonists, type IA antidysrhythmics), myocardial ischemia, dysrhythmia, or infarction (cocaine). Because many overdoses are mixed overdoses, the distinction between cardiogenic pulmonary edema and ARDS is often difficult to establish by physical examination and requires invasive monitoring techniques.
Although the treatments for cardiogenic pulmonary edema and ARDS have many similarities, critical aspects of the therapy differ; therefore, an accurate diagnosis must be established. Most diagnostic tests are not helpful in differentiating between the 2 diseases. Physical examination reveals the presence of crackles with both entities. An S3 gallop, if present, suggests a cardiac cause, but its absence does not establish the diagnosis of ARDS. In both entities, the arterial blood–gas analysis demonstrates hypoxia and chest radiography shows perihilar, basilar, or diffuse alveolar infiltrates. However, the presence of “vascular redistribution” on chest radiography is suggestive of a cardiogenic etiology; a normal-sized heart is more commonly associated with ARDS, whereas an enlarged heart is more typical of cardiogenic pulmonary edema. The diagnostic tests that are useful in establishing the correct diagnosis include echocardiography, transcutaneous bioimpedance, pulmonary artery catheter pressure measurements, and radionuclide ventriculography (“gated-pool” scan). Although the radionuclide scan accurately measures cardiac output, it is not routinely available in the emergency department or intensive care unit and usually requires the transport of a critically ill patient to the nuclear medicine suite. Although echocardiography is easily performed as a portable “bedside” technique, it is less sensitive and less specific for determinations of cardiac output. Therefore, the most definitive diagnostic procedure in the emergency setting is the insertion of a pulmonary artery catheter for hemodynamic monitoring. Cardiogenic pulmonary edema results from an elevated left-atrial filling pressure (elevated pulmonary-capillary wedge pressure) and a decreased cardiac output. In patients with ARDS, the pulmonary artery wedge pressure and the cardiac output are normal. Although not specifically well investigated in poisoning, experiences with transcutaneous bioimpedance measurements of cardiac output show promise for this portable noninvasive technique.10,27,75,79
The basic treatment for ARDS is supportive care while the xenobiotic is eliminated and healing occurs in the pulmonary capillaries.3,61 The most important specific therapeutic intervention in patients with ARDS requiring mechanical ventilation involves the use of low tidal-volume ventilation (≤6 mL/kg predicted body weight).1,43,112
This results in reduced airway pressures, which seem to decrease the chance for alveolar distension and subsequent injury. The efficacy of jet ventilators or membrane oxygenators is inadequately studied. Some studies suggest a potential role for extracorporeal membrane oxygenation in the treatment of ARDS.56 Positive end-expiratory pressure (PEEP), which likely derives its benefit from keeping alveoli open, is considered an essential component in the management of ARDS.31,43
Positive end-expiratory pressure should be maintained in the range of 5 to 12 cm H2O, to maintain a PO2 of at least 55 mm Hg, or an oxygen saturation of 88%, with an inspired oxygen concentration of ≤40%. Although in cases of grave lung injury, higher PEEP settings are often required, they come with an increased incidence of pneumothorax or hypotension. Also, while an increase in PEEP typically results in a modest increase in PO2, it causes a larger decrease in venous return and decreased cardiac output. Therefore, with each change in PEEP, the resulting increase (or perhaps decrease) in oxygen delivery to the body should be determined.4
A conservative fluid strategy improves oxygenation and decreases length of stay in the intensive care unit without increasing the incidence of shock or the need for hemodialysis. A conservative fluid management strategy is recommended for patients with ARDS who are not in shock, have not been in shock for at least 12 hours, and do not have other reasons to require liberal fluid administration.115
Disorders of hemoglobin oxygen content, and of hemoglobin loading and unloading, result in cellular hypoxia, which, in turn, results in hyperventilation. Anemia is a common complication of the infectious diseases associated with injection drug use. In addition, many xenobiotics result in hemolysis or direct bone marrow suppression. Among the latter group are the metals, lead, benzene, and ethanol. Hemolysis also occurs in individuals exposed to lead, copper, or arsine gas, and in patients with glucose-6-phosphate dehydrogenase deficiency exposed to oxidants (Chap. 20).
The oxygen-carrying capacity of blood declines in almost direct proportion to hemoglobin content,106 as seen in Fig. 28–1. As shown in Fig. 28–1A, under most normal conditions the dissolved oxygen content of the blood contributes little; thus, the last portion of the equation can be eliminated. Anemia resulting in a decrease of the hemoglobin content to 7.5 g/dL (a hematocrit of ∼22%) decreases the oxygen content of the blood to about 10.2 mL O2/dL (Fig. 28–1B). Because central cyanosis is only visible with a concentration of reduced deoxyhemoglobin of at least 5 g/dL, unless an abnormal hemoglobin concentration is present, anemia significantly impairs oxygen-carrying capacity without the development of this common physical manifestation (Chap. 124).
FIGURE 28–1.
Examples of calculations of the oxygen content of the blood under various conditions.

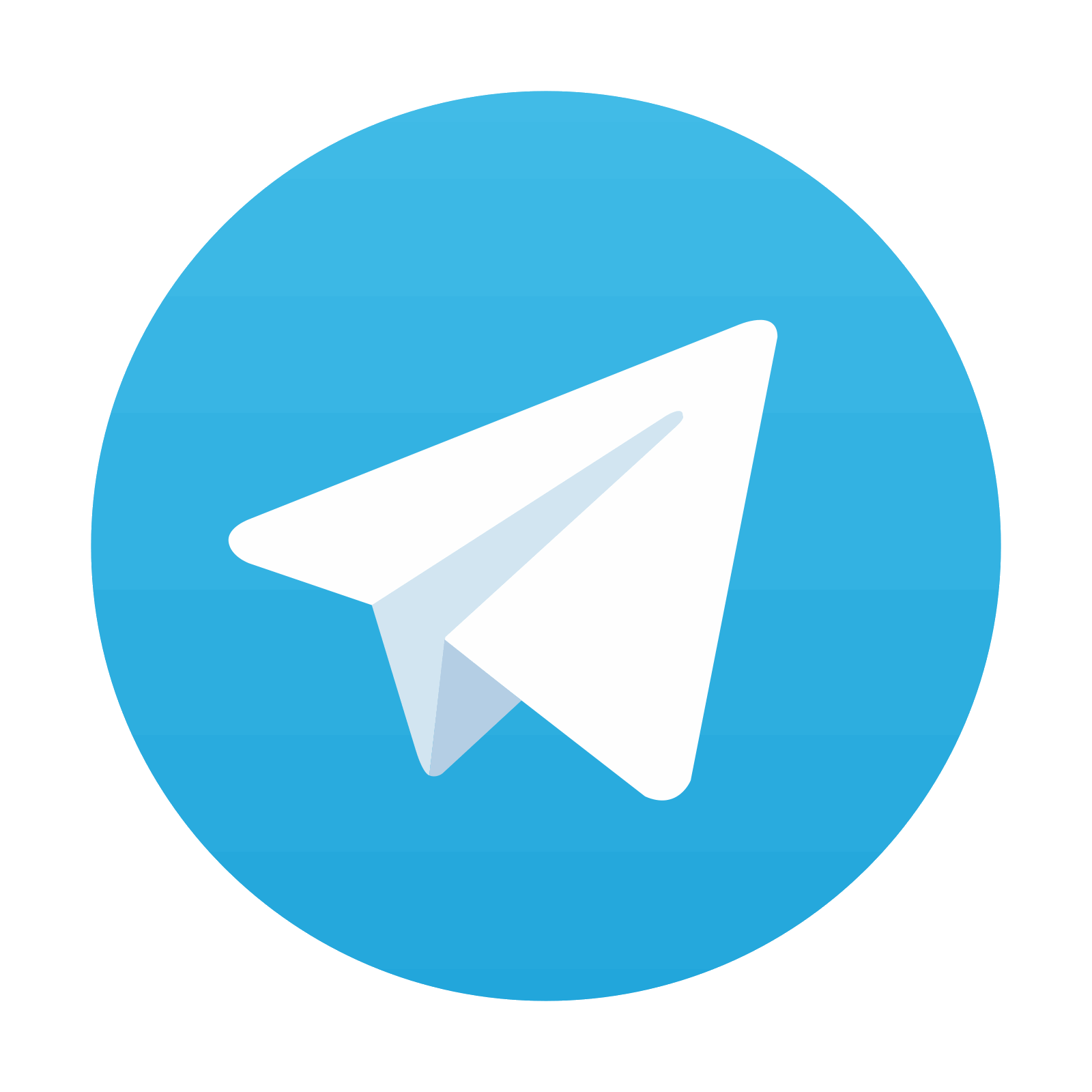
Stay updated, free articles. Join our Telegram channel
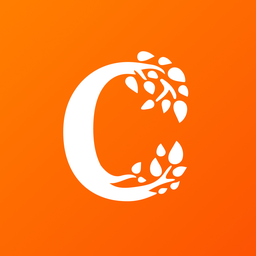
Full access? Get Clinical Tree
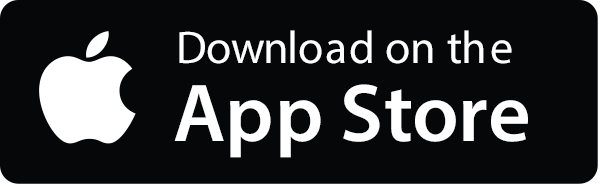
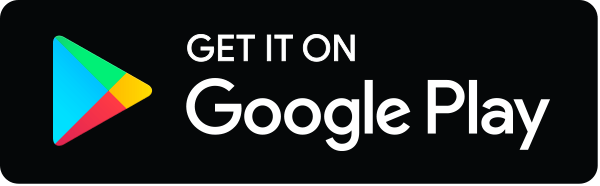
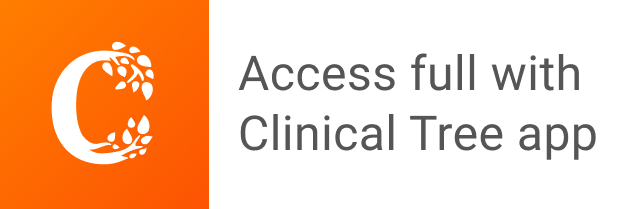