Blood is rightfully considered the vital fluid, because every organ system depends on the normal function of blood. Blood delivers oxygen and other essential substances throughout the body, removes waste products of metabolism, transports hormones from their origin to site of action, signals and defends against threatened infection, promotes healing via the inflammatory response, and maintains the vascular integrity of the circulatory system through the clotting cascade. It also serves as the central compartment in classical pharmacokinetics and thereby comes into direct contact with virtually every systemic xenobiotic that acts on the organism.92 The ease and frequency with which blood is assayed, its central role in functions vital to the organism, and the ability to analyze its characteristics, at first by light microscopy and more recently with molecular techniques, have enabled a detailed understanding of blood that continues to advance the frontiers of molecular medicine.
In addition to transporting xenobiotics throughout the body, blood and the blood-forming organs can at times be directly affected by these same xenobiotics. For example, decreased blood cell production, increased blood cell destruction, alteration of hemoglobin, and impairment of coagulation can all result from exposure to xenobiotics. The response in many cases depends on the nature and quantity of the xenobiotic as well as the capacity of the system to defend against the insult. In other cases, no clear and predictable dose–response relationship can be determined, especially when the interaction involves the immune system. These latter reactions are often termed idiosyncratic, reflecting an incomplete understanding of their causative mechanism. In general, such reactions can often be reclassified when advancing knowledge identifies the characteristics that render the individual vulnerable.
Hematopoiesis is the development of the cellular elements of blood. The majority of the cells of the blood system are classified as either lymphoid (B, T, and natural killer lymphocytes) or myeloid (erythrocytes, megakaryocytes, granulocytes, and monocytes). All of these cells originate from a small common pool of totipotent cells called hematopoietic stem cells. Indeed, the study of this process and its regulation has provided fundamental insight into embryogenesis, stem cell pluripotency, and complex cell-to-cell signaling and interaction.
Marrow spaces within bone begin to form in humans at about the fifth fetal month and become the sole site of granulocyte and megakaryocyte proliferation. Erythropoiesis moves from the liver to the marrow by the end of the last trimester. At birth, all marrow contributes to blood cell formation and is red, containing very few fat cells. By adulthood, the same volume of hematopoietic marrow is normally restricted to the sternum, ribs, pelvis, upper sacrum, proximal femora and humeri, skull, vertebrae, clavicles, and scapulae. Extramedullary hematopoiesis in the liver and spleen reemerges as a compensatory mechanism only under severe stimulation.
Progenitor cells must interact with a supportive microenvironment to sustain hematopoiesis. The hematopoietic stroma consists of macrophages, fibroblasts, adipocytes, and endothelial cells. The extracellular matrix is produced by the stromal cells and is composed of various fibrous proteins, glycoproteins, and proteoglycans, such as collagen, fibronectin, laminin, hemonectin, and thrombospondin. Hematopoietic progenitor cells have receptors to particular matrix molecules. The extracellular matrix provides a structural network to which the progenitors are anchored. As the cells approach maturity, they lose their surface receptors, allowing them to leave the hematopoietic space and enter the venous sinuses. Blood cell release depends on a pressure gradient that drives proliferating, mature cells through endothelial cells lining the sinusoidal spaces.34 This pressure is contained by the surrounding rigid bone cortex and increases under the influence of erythropoietin and granulocyte colony-stimulating factor.
A stem cell is capable of self-renewal, as well as differentiation into a specific cell type. The pluripotent hematopoietic stem cells can therefore continuously replicate while awaiting the appropriate signal to differentiate into either a myeloid or a lymphoid stem cell (Fig. 20–1). Stem cells represent only 1 in 100,000 of the nucleated cells of the bone marrow, and most of these stem cells are usually quiescent. Nevertheless, these relatively few cells are directly responsible for the estimated 200 billion red cells, 150 billion platelets, and 100 billion leukocytes produced each day.34 In response to hemolysis or infection, substantially larger numbers of blood cells are produced.73 Multiple steps are involved in the commitment of less-differentiated cells to more mature cell lines. The final steps in the maturation of erythrocytes alone, for example, involve extensive remodeling, the restructuring of cellular membranes, the accumulation of hemoglobin, and the loss of nuclei and organelles.
Multipotent mesenchymal stem cells capable of differentiating into other tissue lines, including hepatic, renal, muscle, and perhaps neuronal, are also found in the bone marrow.41 Furthermore, tissue-specific stem cells capable of self-renewal reside in numerous other organs and to play a fundamental role in repair and regeneration.21 A variety of strategies have likely evolved to protect the stem cell from injury due to xenobiotics and radiation, and a better understanding of these effects promises to improve our understanding of toxicity and treatment. The hematopoietic stem cell has provided fundamental insights into regenerative biology, and it remains a focus of intense research given the profound implications for organ homeostasis, tissue repair, and gene therapy.59,62
Cytokines are soluble mediators secreted by cells for cell-to-cell communication. Initially termed growth factors, it is now recognized that not all cytokines are growth factors. Cytokines promote or inhibit the differentiation, proliferation, and trafficking of blood cells and their precursors. Importantly, they can also inhibit apoptosis, and their absence therefore results in the self-destruction of unwanted cells. They include growth factors or colony-stimulating factors (CSFs), interleukins, monokines, interferons, and chemokines. At baseline, these act in concert to maintain normal blood counts. In response to antigens or other stimuli, cytokines are released to combat perceived infection. Recombinant cytokines are being used therapeutically in immunocompromised patients, transplant recipients, and those with hepatitis C, anemia, multiple sclerosis, and cancer. They are also being used in clinical toxicology, for example, for the treatment of leukocytopenia that results from colchicine and podophyllum toxicity.29,44
The growth factors are glycoproteins necessary for the differentiation and maturation of individual or multiple cell lines. They fall into 2 families based on their target receptors. The ligands of the cytokine receptor family include growth hormone, interleukin-2 (IL-2), macrophage colony-stimulating factor–1 (CSF-1), granulocyte-macrophage colony–stimulating factor (GM-CSF), γ-interferon, and granulocyte colony–stimulating factor (G-CSF), to name a few. The second group, the tyrosine kinase family, includes Kit ligand and insulinlike growth factor-1 receptor (IGF-1R), a member of the insulin family.
Using monoclonal antibody technology, cell-surface antigens are used to characterize cell types. The cluster designation (CD) nomenclature was introduced by immunologists to ensure a common language when confronted with multiple antibodies to the same leukocyte cell-surface molecule, and hundreds of such unique molecules have been classified.110 For example, the CD34 antigen is a 115-kilodalton (kDa) highly glycosylated transmembrane protein that is selectively expressed by primitive multipotent hematopoietic stem cells shortly after activation, but is absent from mature T and B lymphocytes.64 Advances in genomics and proteomics now complement the immunologic designation, but the ability to subtype blood cells phenotypically has transformed the approach to the leukemias, autoimmune disease, transplantation medicine, and thromboembolic disease.
The human leukocyte antigen (HLA) system denotes the major histocompatibility complex in humans, a group of genes involved in antigen presentation to T lymphocytes, the complement system, cancer surveillance, and autoimmune disease. The rich variation in HLA genes, with more than 100 known alleles at 6 loci, may reflect selective evolutionary pressure to deter individuals with similar HLA from mating. Such sexual selection enhancing diversity would protect a population from coevolving pathogens that attempted to mimic a specific human epitope and avoid immune attack. Indeed, humans appear to be able to distinguish and preferentially choose partners with HLA alleles different from their own using smell, promoting heterozygous diversity.68 Exposure to blood transfusions, pregnancy, and organ grafts can generate antibodies against non-self HLAs.
Aplastic anemia is characterized by pancytopenia on peripheral smear, a hypocellular marrow, and delayed plasma iron clearance. Severe aplastic anemia denotes a granulocyte count of less than 500 cells/mm3, a platelet count of less than 20,000/mm3, and a reticulocyte count of less than 1% after correction for anemia. Following acute insult and depletion of extracirculatory reserves, cell line counts fall at a rate inversely proportional to their life span: granulocytes (circulating half-life 6–12 hours) disappear within days, platelets (life span of 7–10 days) decline by half in about one week, while erythrocytes (normal life span 120 days) decline over weeks in the absence of bleeding or hemolysis. Approximately 1,000 new cases of aplastic anemia are diagnosed yearly in the United States. The incidence is 2 to 3 times higher in Asia, perhaps because of a combination of genetic, environmental, and infectious factors.108 Aplastic anemia may be inborn (as in Fanconi anemia) or acquired. A variety of xenobiotics such as benzene, pesticides, and chloramphenicol are associated with acquired aplastic anemia (Table 20–1),20,109 but causality is uncertain given uncertain case ascertainment and other biases. Epidemiologic studies demonstrate that specific causes are identified in relatively few cases.52
Analgesics | Antirheumatics |
Acetaminophen | Gold salts |
Diclofenac | Methotrexate |
Dipyrone | D-Penicillamine |
Indomethacin | Antithyroids |
Phenylbutazone | Propylthiouracil |
Salicylates | Chemotherapeuticsa |
Antidysrhythmics | Adriamycina |
Tocainide | Antimetabolites |
Antiepileptics | Colchicine |
Carbamazepine | Daunorubicina |
Felbamate | Mustards |
Levetiracetam | Vinblastine |
Antihistamines | Vincristine |
Cimetidine | Diuretics |
Antimicrobials | Acetazolamide |
Albendazole | Metolazone |
Chloramphenicol | Occupational |
Mefloquine | Arsenica |
Penicillin | Benzenea |
Zidovudine | Cadmium |
Antiplatelets | Pesticides |
Ticlopidine | Radiationa |
Antipsychotics | |
Chlorpromazine | |
Clozapine |
Generally speaking, the majority of cases of so-called idiosyncratic aplastic anemia are caused by autoimmune attack on CD34+ hematopoietic stem cells. Following an exposure to an inciting antigen, cytotoxic T cells secrete interferon-γ and tumor necrosis factor α, which destroy progenitor cells, eventually depleting mature leukocytes, erythrocytes, and platelets in circulation.109 As with other autoimmune diseases, certain HLA patterns are associated with a genetic predisposition for the condition, namely the HLA DR2. Clozapine-induced agranulocytosis is associated with the HLA B38, DR4, and DQ3 haplotypes.74 Immunosuppressive therapy or allogenic stem cell transplantation allows recovery of hematopoiesis, and survival is now expected.108
It is important to distinguish immunologic xenobiotic-induced aplastic anemia from the direct myelotoxic effects of radiation and chemotherapy. Following exposure to ionizing radiation, a pancytopenia ensues as a result of injury to stem and progenitor cells. Although atom bomb survivors rarely developed aplastic anemia,49 fractionated whole body radiation of at least 10 Gy is used to intentionally destroy bone marrow stem cells prior to hematopoietic stem cell transplantation. Cancer chemotherapy is often dosed to the endpoint of reversible hematopoietic toxicity. Vacuolated pronormoblasts are found in the bone marrow when aplasia is due to a myelotoxic drug, and treatment consists primarily of stopping the offending xenobiotic while supporting cell lines and preventing infection and bleeding. Other nonimmunologic mechanisms of aplasia are identified in specific cases. For example, the severe pancytopenia that occasionally occurs following 5-fluorouracil therapy is caused by a deficiency of dihydropyrimidine dehydrogenase present in 3% to 5% of whites.104
The erythron is often considered to be a single yet dispersed tissue, defined as the entire mass of erythroid cells from the first committed progenitor cell to the mature circulating erythrocyte (red blood cell). This functional definition emphasizes the integrated regulation of the erythron, both in health and disease. The primary function of the erythron is to transport molecular oxygen throughout the organism. To accomplish this, adequate numbers of circulating erythrocytes (nearly half of the blood by volume) must be maintained. These erythrocytes must be able to preserve their structure and flexibility to circuit repeatedly through the microcirculation and to resist oxidant stress accumulated during their life span.84 The erythrocyte also plays a key role in modulating vascular tone. Interactions between oxyhemoglobin and nitric oxide help match vasomotor tone to local tissue oxygen demands.30,39,43,67,91
Homeostasis of erythron proliferation is primarily maintained by the equilibrium between stimulation via the hormone erythropoietin and apoptosis controlled by 2 receptors, Fas and FasL, expressed on the membranes of erythroid precursors. At the other extreme, erythrocytes are culled from the circulation at the end of their life span primarily by the action of the spleen. With age, erythrocytes become less able to negotiate the narrow red pulp passages in the spleen and are phagocytosed by macrophages. By filtering out these senescent cells, the spleen minimizes entrapment in the microvasculature of other organs and prevents spillage of intracellular contents including hemoglobin into the intravascular circulation.
Erythropoietin (EPO) is a glycoprotein hormone of molecular weight 34,000 Da that is produced in the epithelial cells lining the peritubular capillaries of the kidney. Anemia and hypoxemia stimulate its synthesis. Erythropoietin receptors are found in human erythroid cells, megakaryocytes, and fetal liver. Erythropoietin promotes erythroid differentiation, the mobilization of marrow progenitor cells, and the premature release of marrow reticulocytes. The cell most sensitive to EPO is a cell between the erythroid colony–forming unit (CFU-E) and the proerythroblast. The absence of EPO results in DNA cleavage and erythroid cell death.
The mature erythrocyte is a highly specialized cell, designed primarily for oxygen transport. Accordingly, it is densely packed with hemoglobin, which constitutes approximately 90% of the dry weight of the erythrocyte. During maturation, the erythrocyte loses its nucleus, mitochondria, and other organelles, rendering it incapable of synthesizing new protein, replicating, or using the oxygen being transported for oxidative phosphorylation. Its metabolic repertoire is severely limited and largely restricted to a few pathways (described below under Metabolism). In general, the enzymatic pathways are those required for optimizing oxygen and carbon dioxide exchange, transiting the microcirculation while maintaining cellular integrity and flexibility, and resisting oxidant stress on the iron and protein of the cell. The characteristic biconcave discocyte shape is dynamically maintained, increasing membrane surface-to-cell volume. This shape decreases intracellular diffusion distances to the cell membrane and allows plastic deformation when squeezing through the microcirculation. The shape is the net sum of elastic and electrostatic forces within the membrane, surface tension, and osmotic and hydrostatic pressures. The cell membrane contains globular proteins suspended within the phospholipid bilayer. The major blood group antigens are carried on membrane ceramide glycolipids and proteins, particularly glycophorin A and the Rh proteins. Membrane proteins generally serve to maintain the structure of the cell, to transport ions and other substances across the membrane, or to catalyze a limited number of specific chemical reactions for the cell.
The cell membrane is coupled to, and interacts dynamically with, the cytoskeleton, allowing changes in cell shape such as tank treading or rotation of the membrane relative to the cytoplasm. This cytoskeleton consists of a hexagonal lattice of proteins, especially spectrin, actin, and protein 4.1, which interact with ankyrin and band 3 in the membrane to provide a strong but flexible structure to the membrane. Other essential structural proteins include tropomyosin, tropomodulin, and adducin. The absence or abnormalities of these proteins results in abnormal erythrocyte shapes such as spherocytes and elliptocytes.
Many specialized transport proteins are embedded in the erythrocyte membrane. These include anion and cation transporters, glucose and urea transporters, and water channels. The erythrocyte membrane is relatively impermeable to ion flux. Band 3 anion-exchange protein plays an important role in the chloride–bicarbonate exchanges that occur as the erythrocyte moves between the lung and tissues. Glucose, the sole source of energy of the erythrocyte, crosses the membrane by facilitated diffusion mediated by a transmembrane glucose transporter. Sodium-potassium adenosine triphosphatase (Na+,K+,ATPase) maintains the primary cation gradient by pumping sodium out of the erythrocyte in exchange for potassium.
At least 50 membrane-bound or membrane-associated enzymes are known to exist in the human erythrocyte. Acetylcholinesterase is an externally oriented enzyme whose role in the function of the erythrocyte remains obscure. Its function is inhibited by certain xenobiotics, most notably the organic phosphorus compounds, and it is conveniently assayed as a marker for such exposures (Chap. 110).
Lacking mitochondria and the ability to generate adenosine triphosphate (ATP) using molecular oxygen, the mature erythrocyte has a severely limited repertoire of intermediary metabolism compared to most mammalian cells. Having lost its nucleus, ribosomes, and translational apparatus, new enzymes cannot be synthesized and existing enzymes decline in function over the lifetime of the cell. Fortunately, the metabolic demands of the erythrocyte are usually modest, but under conditions of stress that capacity can be overwhelmed, especially among senescent cells. The greatest expenditure of energy under physiologic conditions is for the maintenance of transmembrane gradients and for the contraction of cytoskeletal elements. However, oxidant stress can put severe strain on the metabolic reserves of the erythrocyte, and lead ultimately to the premature destruction of the cell, a process termed hemolysis.
Figure 20–2 illustrates the main metabolic pathways and their purpose. Embden-Meyerhof glycolysis is the only source of ATP for the erythrocyte and consumes approximately 90% of the glucose imported by the cell. The reduced nicotinamide adenine dinucleotide (NADH) generated during glycolysis, which would ordinarily be used for oxidative phosphorylation in cells containing mitochondria, is directed toward the reduction of either methemoglobin to hemoglobin by cytochrome b5 reductase, or of pyruvate to lactate. Both pyruvate and lactate are exported from the cell. In response to anemia, altitude, or changes in cellular pH, glycolysis is diverted into the Rapoport-Luebering shunt, generating 2,3-bisphosphoglycerate (2,3-BPG, formerly known as 2,3-diphosphoglycerate or 2,3-DPG) in lieu of ATP. 2,3-bisphosphoglycerate binds to deoxyhemoglobin to modulate oxygen affinity and to allow unloading of oxygen at the capillaries, thereby increasing oxygen delivery considerably. Reduced levels of 2,3-BPG in stored blood result in impaired oxygen delivery following massive transfusion.101
FIGURE 20–2.
Metabolic pathways of the erythrocyte. The main metabolic pathways available to the mature erythrocyte are shown (rectangles). Glucose is imported into the cell, while pyruvate, lactate, and oxidized glutathione (GSSG) are exported. 2,3-BPG = 2,3-bisphosphoglycerate; ADP = adenosine diphosphate; ATP = adenosine triphosphate; cyt b5 red = cytochrome b5 reductase; G6PD = glucose-6-phosphate dehydrogenase; GSH = reduced glutathione; Hb = hemoglobin; NADH = reduced form of nicotinamide adenine dinucleotide (NAD+); NADPH = reduced form of nicotinamide adenine dinucleotide phosphate (NADP+); RBC = red blood cell (erythrocyte).

As an alternative to glycolysis, glucose is diverted to the hexose monophosphate shunt during times of oxidant stress. This pathway results in the generation of reduced nicotinamide adenine dinucleotide phosphate (NADPH), which the erythrocyte uses to maintain reduced glutathione. Reduced glutathione, in turn, inactivates oxidants and protects the sulfhydryl groups of hemoglobin and other proteins. The initial, rate-limiting step of this pathway is controlled by glucose-6-phosphate dehydrogenase (G6PD). Accordingly, cells deficient in this enzyme are less able to maintain reduced glutathione, and are vulnerable to irreversible damage under oxidant stress. The consequences of this deficiency are discussed in greater detail below under Glucose-6-Phosphate Dehydrogenase Deficiencies.
The erythrocyte also contains enzymes to synthesize glutathione (γ-glutamyl-cysteine synthetase and glutathione synthetase), to convert CO2 to bicarbonate ion (carbonic anhydrase I), to remove pyrimidines resulting from the degradation of RNA (pyrimidine 5′-nucleotidase), to protect against free radicals (catalase, superoxide dismutase, glutathione peroxidase), and to conjugate glutathione to electrophiles (ρ-glutathione-S-transferase).
Hemoglobin, the major constituent of the cytoplasm of the erythrocyte, is a conjugated protein with a molecular weight of 64,500 Da. To put things in perspective, the typical adult man has approximately 75 mL/kg of blood containing 15 g/100 mL of hemoglobin, or nearly 1 kg of hemoglobin. His 0.3-kg heart must pump this entire mass of hemoglobin every minute at rest, which is a substantial work expenditure. One molecule is composed of 4 protein (globin) chains, each attached to a prosthetic group called heme. Heme contains an iron molecule complexed at the center of a porphyrin ring. The globin chains are held together by noncovalent electrostatic attraction into a tetrahedral array. Hemoglobin is so efficient at binding and carrying oxygen that it enables blood to transport nearly 100 times as much oxygen as could be carried by plasma alone (Chap. 28). In addition, the capacity of hemoglobin to modulate oxygen binding under different conditions allows adaptation to a wide variety of environments and demands. Three complex and integrated pathways are required for the formation of hemoglobin: globin synthesis, protoporphyrin synthesis, and iron metabolism.
The protein chains of hemoglobin are produced with information from 2 different genetic loci. The α-globin gene cluster spans 30 kb on the short arm of chromosome 16, and codes for 2 identical adult α-chain genes, as well as the ζ-chain, an embryonic globulin. The β cluster is 50 kb on chromosome 11, and codes for the 2 adult globins β and δ, as well as 2 nearly identical γ chains expressed in the fetus and an embryonic globulin ε. The expression of genes in each family changes during embryonic, fetal, neonatal, and adult development. Until 8 weeks of intrauterine life, ε, ζ, γ, and α chains are produced and assembled in various combinations in yolk sac–derived erythrocytes. With the shift in erythropoiesis from yolk sac to fetal liver and spleen, embryonic hemoglobin disappears, and the α and γ globin chains are paired into fetal hemoglobin (HbF = α2γ2). Erythrocytes containing HbF have a higher O2 affinity than those containing adult hemoglobin, which is important for oxygen transfer across the placenta into the relatively hypoxic uterine environment. Beginning shortly before birth, expression at the β cluster shifts to the β and δ globins. The predominant adult hemoglobin is termed hemoglobin A (α2β2), whereas approximately 2.5% of normal adult hemoglobin is in the form of hemoglobin A2 (α2δ2). The thalassemias, a group of inherited disorders, result from defective synthesis of one or more of the globin chains. Clinically this results in a hypochromic, microcytic anemia.
Heme is the iron complex of protoporphyrin IX. Protoporphyrin IX is a tetramer composed of 4 porphyrin rings joined in a closed, flat-ring structure. The IX designation refers to the order in which it was first synthesized in Hans Fischer’s laboratory. Of the 15 possible isomers, only protoporphyrin IX occurs in living organisms. Technically, only iron complexes with the iron in the Fe2+ state can be called heme, but the term is commonly used to refer to the prosthetic group of metalloproteins such as peroxidase (ferric) and cytochrome c (both ferric and ferrous), whether the iron is in the Fe2+ or Fe3+ state. The terms “hemiglobin” and “ferrihemoglobin” are synonymous with methemoglobin but rarely used. All animal cells can synthesize heme, with the notable exception of mature erythrocytes. Hemoproteins are involved in a multitude of biologic functions, including oxygen binding (hemoglobin, myoglobin), oxygen metabolism (oxidases, peroxidases, catalases, and hydroxylases), and electron transport (cytochromes), as well as metabolism of xenobiotics (cytochrome P450 family). Erythroid cells synthesize 85% of total body heme, with the liver synthesizing most of the balance. Hemoglobin is the most abundant hemoprotein, containing 70% of total body iron.
The first step in the synthesis of heme takes place in the mitochondrion and is the condensation of glycine and succinyl-coenzyme A (CoA) to form 5-aminolevulinic acid (ALA) (Fig. 20–3). The formation of 5-ALA is catalyzed by aminolevulinic acid synthase (ALAS), the rate-limiting step of the pathway. The rate of heme synthesis is closely controlled, given that free intracellular heme is toxic and that this first step is essentially irreversible.
Of the 2 isoforms of ALAS known to exist in mammals, erythroid cells express the ALAS2 isoform, which resides on the X chromosome. Comparatively more is known about ALAS1 (chromosome 3), a housekeeping gene with a short half-life expressed ubiquitously, allowing the synthesis of cellular and mitochondrial hemoproteins. Aminolevulinic acid synthase (ALAS1) activity is induced by many factors, which can increase its expression by 2 orders of magnitude. Moreover, it is strongly inhibited by heme in a classical negative feedback fashion. Aminolevulinic acid synthase (ALAS2) is constitutively expressed at very high concentrations in erythroid precursors, allowing sustained synthesis of heme during erythropoiesis. Pyridoxal phosphate (active vitamin B6) serves as a cofactor to both isoforms of ALAS. The clinical consequences of pyridoxine deficiency include a hypochromic, microcytic anemia, iron overload, and neurologic impairment.
The next step in the synthesis of hemoglobin is the formation of the monopyrrole porphobilinogen via the condensation of 2 molecules of ALA. The subsequent steps in heme synthesis involve the condensation of 4 molecules of porphobilinogen into a flat ring, which is transported back into the mitochondrion by an unknown mechanism. The final step is the insertion of iron into protoporphyrin IX, a reaction that is catalyzed by ferrochelatase (also known as heme synthase) to form heme.
Understanding this carefully regulated synthetic pathway is relevant to understanding the laboratory evidence of lead toxicity (Chap. 93), and predicting the response of porphyric patients to a range of xenobiotics. Most steps in the heme biosynthetic pathway are inhibited by lead. ALA dehydratase is the most sensitive, followed by ferrochelatase, coproporphyrinogen oxidase, and porphobilinogen deaminase. As a consequence, ALA is increased in plasma and especially urine. With increasing exposure, ferrochelatase inhibition coupled with iron deficiency causes zinc protoporphyrin to accumulate in erythrocytes, which can easily be detected by fluorescence. Coproporphyrinogen III also appears in the urine. Historically, these effects have served as the basis for a number of tests of lead exposure.
The porphyrias are a group of disorders resulting from an inherited deficiency of any given enzyme that follows ALAS on the heme biosynthetic pathway. As such, when ALAS activity outpaces the activity of the deficient enzyme, the rate-limiting step shifts downstream, and intermediate metabolites accumulate. These metabolites cause characteristic neuropsychiatric symptoms and palsies (caused by ALA and porphobilinogen), and cutaneous reactions, including photosensitivity (due to the fluorescence of the porphyrins). For example, porphobilinogen is excreted in large quantities by patients with acute intermittent porphyria (porphobilinogen deaminase deficiency), and the urine darkens with exposure to air and light due to oxidation to porphobilin and to nonenzymatic assembly into porphyrin rings. A variety of xenobiotics precipitate crises in susceptible individuals, by inducing ALAS1 and overloading the deficient enzyme.100 The molecular mechanisms that allow xenobiotics to induce the ALAS1 gene closely resemble those accounting for induction of the cytochrome P450 (CYP) genes, which also require heme synthesis. The xenobiotic typically interacts with either the constitutive androstane receptor (CAR) or the pregnane X receptor (PXR), the 2 main so-called orphan nuclear receptors.107 These transcriptional factors are DNA-binding proteins that induce the expression of a range of drug metabolizing and transporting genes in response to the presence of a xenobiotic and are termed “xenosensors.” When activated, they associate with the 9-cis retinoic acid xenobiotic receptor (RXR), and then attach to enhancer sequences near the apoCYP or ALAS1 genes to enhance transcription.81 The multifunctional inducers capable of activating a wide range of hepatic enzymes are therefore extremely porphyrogenic and include phenobarbital, phenytoin, carbamazepine, and primidone. Furthermore, because CYP 3A4 and 2C9 represent nearly half of the hepatic CYP pool, inducers of these isoforms also stimulate heme synthesis and induce porphyric crises. Examples include the anticonvulsants; nifedipine; sulfamethoxazole; rifampicin; ketoconazole; and the reproductive steroids progesterone, medroxyprogesterone, and testosterone. Glucocorticoids, on the other hand, despite binding to PXR, suppress ALAS1 induction and translation, and they are not porphyrogenic.100
At equilibrium, approximately 1 to 2 mg of iron is absorbed from the diet, and a similar amount is shed from the intestinal epithelium each day. Unless appropriately chelated, free iron not bound by transport or storage proteins can generate harmful oxygen free radicals that damage cellular structures and metabolism (Chaps. 10 and 45). For this reason, serum iron circulates bound to a transfer protein, transferrin, and is stored in the tissues using ferritin (Fig. 20–4). Whereas each molecule of transferrin can bind 2 iron atoms, ferritin has a large internal cavity, approximately 80 Å in diameter, that can hold up to 4500 iron atoms per molecule. The amount of iron transported through plasma depends on total-body iron stores and the rate of erythropoiesis. Only about one-third of the iron-binding sites of circulating transferrin are normally saturated, as demonstrated by the usual serum iron content of 60 to 170 mcg/dL (10–30 μmol/L) as compared to the total iron-binding capacity of 280 to 390 mcg/dL (50–70 μmol/L).
FIGURE 20–4.
The iron cycle. The flow of iron is indicated by arrows, and average daily rates of flux are shown for an adult. Absorption at the duodenal enterocyte is tightly controlled, because there are few physiologic processes to regulate losses (other than pregnancy and menstruation). Atomic iron is absorbed via the divalent metal transporter 1, and heme-bound iron via heme carrier protein 1. Hepcidin is an important circulating peptide inhibitor of ferroportin, thereby limiting absorption when stores are adequate. Iron content (either atomic or heme bound) of specific organs or compartments is shown in milligrams. The largest stores of atomic iron are contained by ferritin, mostly in liver and spleen macrophages, and erythrocyte precursors in the marrow. Only a relatively small amount circulates in the plasma bound to transferrin, which is usually only partially saturated. EPO = erythropoietin; RBC = red blood cell.

Only transferrin can directly supply iron for hemoglobin synthesis. The iron–transferrin complex binds to transferrin receptors on the surface of developing erythroid cells in bone marrow. Iron in the erythroid cell is used for hemoglobin synthesis or is stored in ferritin.
The absorption of nonheme, free ferrous iron from the diet occurs via the divalent metal transporter 1 of the duodenal enterocyte, which then passes it into the circulation via ferroportin. Iron then circulates in the ferric form bound to transferrin.46 Dietary iron complexed with heme can also be absorbed via the heme carrier protein-1, which transports either iron or zinc protoporphyrin into the enterocyte.61 The iron may then be freed by microsomal heme oxygenase and follow the transport of atomic iron, or perhaps heme itself can be transferred to the circulation via specific export proteins and circulate bound to its carrier protein, hemopexin.5
When the erythrocyte is removed from the circulation by splenic macrophages, heme is degraded by heme oxygenase to carbon monoxide and biliverdin, and the iron extracted. Some iron remains in macrophages in the form of ferritin or hemosiderin. Most is delivered again by ferroportin back to the plasma and bound to transferrin.
Iron homeostasis is largely regulated at the level of absorption, with little physiologic control over its rate of loss. Excess absorption relative to body stores is the hallmark of hereditary hemochromatosis. The iron regulatory hormone hepcidin produced by the liver plays a central role in iron control, including the anemia of chronic disease caused by inflammatory signals.46 Hepcidin is a 25–amino acid peptide that senses iron stores and controls the ferroportin-mediated release of iron from enterocytes, macrophages, and hepatocytes. The liver is an important reservoir of iron because it can store considerable amounts of iron taken from portal blood and release it when needed.
The evolutionary transition of organisms from anaerobic to aerobic life allowed the liberation of 18 times more energy from glucose. Vertebrates developed 2 important systems to overcome the relatively small quantities of oxygen dissolved in aqueous solutions under atmospheric conditions: the circulatory system and hemoglobin. The circulatory system allows delivery of oxygen and removal of carbon dioxide throughout the organism. Hemoglobin plays an essential role in the transport and exchange of both gases. Moreover, the interactions between these gases and hemoglobin are directly linked in a remarkable story of molecular evolution. Understanding this interplay allowed early insight into protein conformation and the importance of allosteric interactions between molecules.
The binding of each oxygen molecule to atomic iron in any of the 4 heme molecules results in conformational changes that affect binding of oxygen at the remaining sites. This phenomenon is known as cooperativity, and it is necessary for the transport of relatively large quantities of oxygen and for the unloading of most of this oxygen at tissue sites. Cooperativity results from the intramolecular interactions of the tetrameric hemoglobin, and is expressed in the sigmoidal shape of the oxyhemoglobin dissociation curve (Fig. 28–2). Conversely, the monomeric myoglobin has a hyperbolic oxygen binding curve. The partial pressure of oxygen at which 50% of the oxygen-binding sites of hemoglobin are occupied is about 26 mm Hg, in contrast to about 1 mm Hg for myoglobin. Moreover, hemoglobin is nearly 100% saturated at partial oxygen pressures of about 100 mm Hg in the pulmonary capillaries, transporting 1.34 mL of oxygen per gram of hemoglobin A. About one-third of this oxygen can be unloaded under normal conditions at tissue capillaries with partial oxygen pressures around 35 mm Hg. The proportion unloaded rises during exercise and sepsis, as well as with xenobiotics that uncouple oxidative phosphorylation. Elite athletes can extract up to 80% of the available oxygen under conditions of maximal aerobic effort.
The oxygen reserve, however, is only one of the benefits of the large quantity of hemoglobin in circulation. The ability of hemoglobin to buffer the acid equivalent of CO2 in solution is equally vital to respiratory physiology, because it allows the removal of large quantities of CO2 from metabolically active tissues with minimal changes in blood pH. Hemoglobin is by far the largest buffer in circulation, accounting for 7 times the buffering capacity of the serum proteins combined (28 versus 4 mEq H+/L of whole blood). For every 1 mole of oxygen unloaded in the tissue, about 0.5 mole of H+ is loaded onto hemoglobin.
The linked interaction between oxygen and carbon dioxide transport can be first considered from the perspective of oxygen binding to hemoglobin. The affinity of oxygen for hemoglobin is directly affected by pH, which is a function of the CO2 content of the blood. The oxyhemoglobin dissociation curve shifts to the left in lungs, where the level of carbon dioxide, and thus carbonic acid, are kept relatively low as a result of ventilation, an effect that promotes oxygen binding. The curve shifts to the right in tissues where cellular respiration increases CO2 concentrations and lowers pH. This phenomenon, known as the Bohr effect, promotes the uptake of oxygen in the lungs and the release of oxygen at tissue sites.
From the perspective of carbon dioxide transport, hemoglobin also plays an essential albeit indirect role. Carbon dioxide dissolves into plasma, and is slowly hydrated to carbonic acid, which dissociates to H+ and HCO3– (pKa 6.35). The speed of the hydration reaction is accelerated from about 40 seconds to 10 ms by the abundant enzyme carbonic anhydrase located within the erythrocyte. Most carbon dioxide collected at the tissues diffuses into erythrocytes, where it becomes H+ and HCO3–. This HCO3– is then rapidly transported back to the serum in exchange for chloride ion via the band 3 anion exchange transporter located in the erythrocyte membrane, thereby shifting serum Cl– into the erythrocyte (the chloride shift). The hydrogen ion is accepted by hemoglobin, largely at the imidazole ring of histidine residues, which have a pKa of about 7.0. A small amount of CO2 reacts directly with the amino terminal of the globin chains to form carbamino residues (HbNHCOO–). Thus, most of the transported carbon dioxide is transformed by the erythrocyte into bicarbonate ion that is returned to the serum and hydrogen ion that is buffered by hemoglobin. Each liter of venous blood typically carries 0.8 mEq dissolved CO2 + 16 mEq HCO3– in the plasma, and 0.4 mEq dissolved CO2 + 4.6 mEq HCO3– + 1.2 mEq HbNHCOO– in the erythrocyte (a total of 23 mEq CO2, equivalent to 510 mL CO2/L blood). Although two-thirds of the total CO2 content is ultimately carried in the plasma, nearly all of the bicarbonate is generated within erythrocytes. In the capillaries of the lungs, the reverse reactions occur to eliminate CO2. Because deoxyhemoglobin is better able to buffer hydrogen ions, the release of oxygen from hemoglobin at the tissues facilitates the uptake of carbon dioxide into venous blood. This effect is known as the Haldane effect. In fact, 1 L of venous blood at 70% oxygen saturation can transport an additional 20 mL of CO2 compared to arterial blood, which is nearly 100% saturated. Both the Bohr and Haldane effects have important consequences at the extremes of acid–base perturbations, as occurs in a number of poisonings that interfere with oxygen metabolism.
Finally, in addition to inactivating nitric oxide, hemoglobin also reversibly binds nitric oxide as S-nitrosohemoglobin, thereby playing an important role in the regulation of microvascular circulation and oxygen delivery. The ability of hemoglobin to vasodilate the surrounding microvasculature in response to oxygen desaturation using nitric oxide provides new insight into oxygen delivery and may be pivotal in such disorders as septic shock, pulmonary hypertension, and senescence of stored red blood cells.94
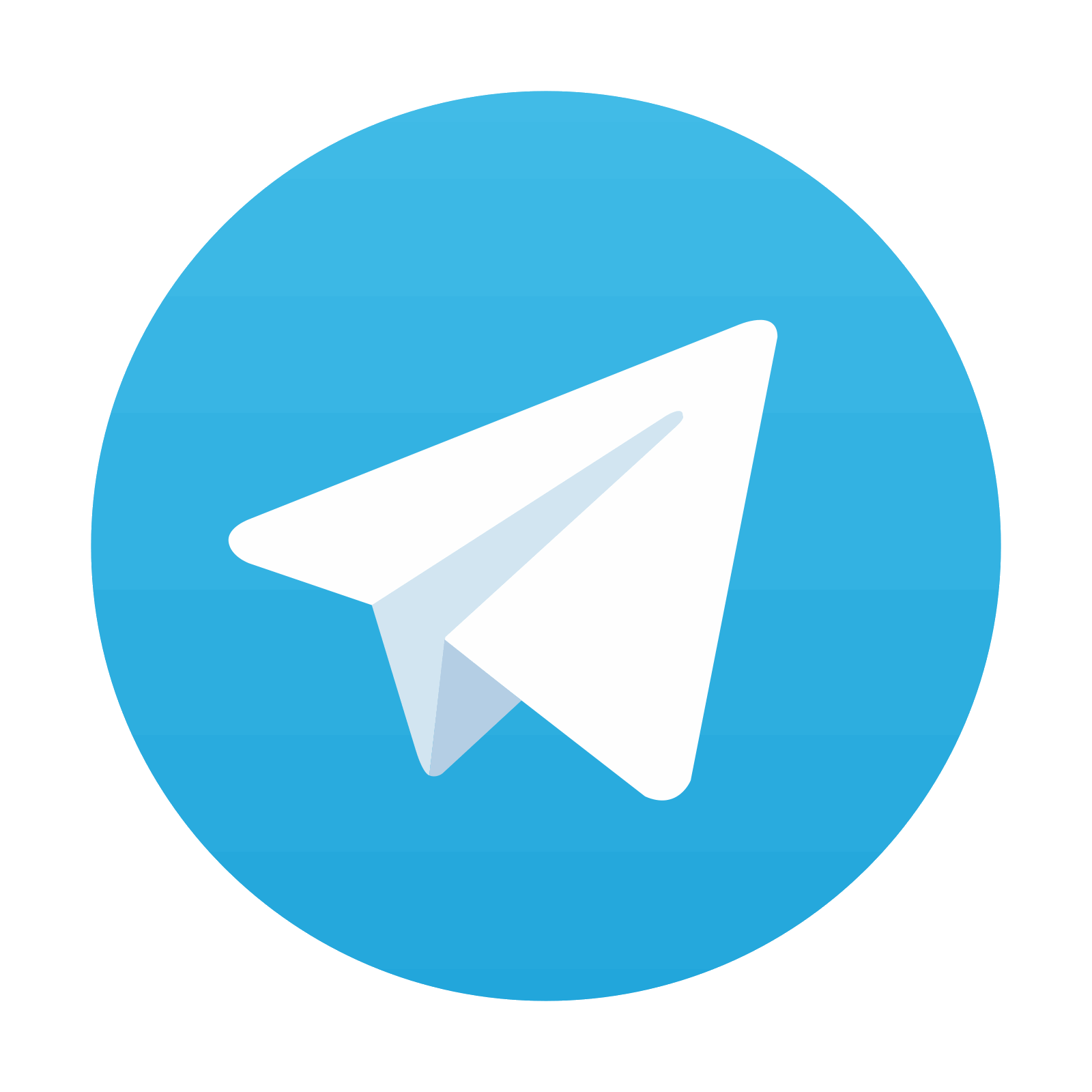
Stay updated, free articles. Join our Telegram channel
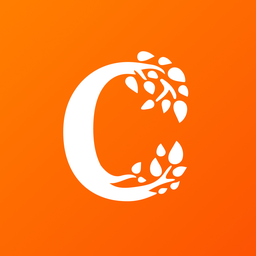
Full access? Get Clinical Tree
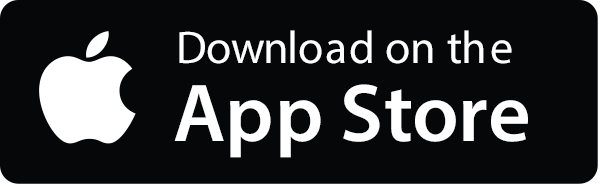
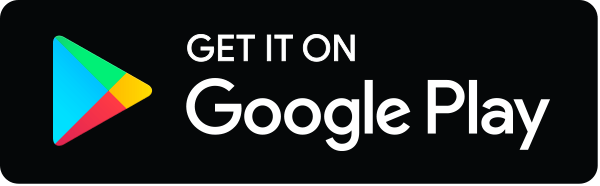