Chemistry is the science of matter; it encompasses the structure, physical properties, and reactivities of atoms and their compounds. In many respects, toxicology is the science of the interactions of matter with living entities; chemistry and toxicology are therefore intimately linked. The study of the principles of inorganic, organic, and biologic chemistry offers important insight into the mechanisms and clinical manifestations of xenobiotics and poisoning.5 This chapter reviews many of these tenets and provides relevance to the current practice of medical toxicology.
Matter includes the substances of which everything is made. Elements are the foundation of matter, and all matter is made from one or more of the known elements. An atom is the smallest quantity of a given element that retains the properties of that element. Atoms consist of a nucleus, incorporating protons and neutrons, coupled with its orbiting electrons. The atomic number is the number of protons in the nucleus of an atom, and it is a whole number that is unique for each element. Thus, elements with 6 protons are always carbon, and all forms of carbon have exactly 6 protons. However, although the vast majority of carbon nuclei have 6 neutrons in addition to the protons, accounting for an atomic mass (protons plus neutrons) of 12 (12C), a small proportion of naturally occurring carbon nuclei, called isotopes, have 8 neutrons and a mass number of 14 (14C). This is the reason that the atomic weight of carbon displayed on the periodic table is 12.011, and not 12, as it actually represents the average atomic masses of all isotopes found in nature weighted by their frequency of occurrence. 14C is also a radioisotope, which is an isotope with an unstable nucleus that emits radiation until it achieves a stable state (Chap. 128). The atomic weight, measured in grams per mole (g/mol), also indicates the molar mass of the element. That is, in one atomic weight of matter (12.011 g for carbon) there is one mole (6.022140857 × 1023) of atoms as defined by Avogadro’s constant.
Elements combine chemically to form compounds, which generally have physical and chemical properties that differ from those of the constituent elements. The elements in a compound can be separated only by chemical means that destroy the original compound, as occurs during the burning (ie, oxidation) of a hydrocarbon, a process that releases the carbon principally as carbon dioxide and the hydrogen principally as water (H2O). This important property differentiates compounds from mixtures. Mixtures are combinations of elements or compounds that can be separated by physical means, such as the distillation of petroleum into its hydrocarbon components or the evaporation of seawater to separate water from sodium chloride and other substances. With notable exceptions, such as the elemental forms of many metals or halogens (eg, Cl2), most xenobiotics are compounds or mixtures.
Dmitri Mendeleev, a Russian chemist in the mid-19th century, recognized that when all of the known elements were arranged in order of atomic weight, certain patterns of reactivity became apparent. The result of his work was the Periodic Table of the Elements (Fig. 10–1), which, with some minor alterations, is still an essential tool today. All of the currently recognized elements are represented; those heavier than uranium do not occur in nature. Many of the symbols used to identify the elements refer to the Latin name of the element. For example, silver is Ag, for argentum, and mercury is Hg, for hydrargyrum, literally “silver water.”
The periodicity of the table relates to the electrons that circle the nucleus in discrete orbitals. Although the details of quantum mechanics and electronic configuration are complex, it is important to review some aspects in order to predict chemical reactivity. Orbitals, or quantum shells, represent the energy levels in which electrons exist around the nucleus. The orbitals are identified by various schemes, but the maximum number of electrons each orbital can contain is calculated as 2x2, where x represents the numerical rank order of the orbital. Thus, the first orbital may contain 2 electrons, the second orbital may contain 8, the third may contain 18, and so on. However, the outermost shell (designated by s, p, d, f nomenclature) of each orbital may only contain up to 8 electrons. This is irrelevant through element 20, calcium, because there is no need to fill the third-level or d shells. Even though the third orbital may contain 18 electrons, once 8 are present the 4s electrons dip below the 3d electrons in energy and this shell begins to fill. This occurs at element 21, scandium, and accounts for its chemical properties and those of the other transition elements. Transition elements are chemically defined as elements that form at least one ion with a partially filled subshell of d electrons. The inert gas elements, which are also known as noble gases, have complete outermost orbitals, accounting for their lack of reactivity under standard conditions.
In general, only electrons in unfilled shells, or valence shells, are involved in chemical reactions. This property relates to the fact that the most stable form of an element occurs when the configuration of its valence shell resembles that of the nearest noble gas, found in group 18 on the periodic table. This state can be obtained through the gaining, losing, or sharing of electrons with other elements and is the basis for virtually all chemical reactions.
Broadly, the periodic table is divided into metals and nonmetals. Metals, in their elemental form, are typically malleable solids that conduct electricity, whereas nonmetals are usually dull, fragile, nonconductive compounds (C, N, P, O, S, Se, and the halogens). Metals are found on the left side of the periodic table, and account for the majority of the elements, whereas nonmetals are on the right side. Separating the 2 groups are the metalloids, which fall on a jagged line starting with boron (B, Si, Ge, As, Sb, Te, At). Metalloids have chemical properties that are intermediate between the metals and the nonmetals. Each column of elements is termed a family or group, and each row is a period. Although the table is conceived and organized in periods, trends in the chemical reactivity, and therefore toxicity, typically exist within the groups.
In 1990, the International Union of Pure and Applied Chemistry (IUPAC) replaced the existing numbering schemes for the periodic table columns with a unified and simplified nomenclature. Using this format, which is included in this chapter, the groups are simply incrementally numbered from 1 to 18 from left to right.2
The ability of any particular element to produce toxicologic effects relates directly to one or more of its many physicochemical properties. These properties will, to some extent, be predicted by their location on the periodic table. For example, arsenate will substitute for phosphate in the mitochondrial production of adenosine triphosphate (ATP), creating adenosine diphosphate monoarsenate (Chap. 11). Because this compound is unstable and not useful as an energy source, energy production by the cell fails; in this manner, arsenic interferes with oxidative phosphorylation. The existence of an interrelationship between Ca2+ and either Mg2+ or Ba2+ is predictable, although the actual effects are not. Under most circumstances, Mg2+ is a Ca2+ antagonist, and patients with hypermagnesemia present with neuromuscular weakness caused by the blockade of myocyte calcium channels. Alternatively, Ba2+ mimics Ca2+ and closes Ca2+-dependent K+ channels in myocytes, producing life-threatening hypokalemia. The physiologic relationships among the ions of lithium (Li+), potassium (K+), and sodium (Na+) are also consistent with their chemical similarities (all alkali metals in Group IA). However, the clinical similarity between thallium (thallous) ion (Tl+) and K+ is not immediately predictable. Other than their monovalent nature (ie, 1+ charge), it is difficult to predict the substitution of Tl+ (Group 13, Period 6) for K+ (Group 1, Period 4) in membrane ion channel functions, until the similarity of their ionic radii is known (Tl+, 1.47 Å; K+, 1.33 Å).
An emerging physicochemical property of toxicological interest is particle size. The chemical and toxicologic properties of a given element or molecule change as the particle size is reduced. An entire field of nanotoxicology has emerged to study the adverse health effects of nanoparticles, which are ultrafinely divided particles, ranging from approximately 1 to 100 nm in diameter. Differences in absorption, biodistribution, and structure-activity effects, for example, enhance the toxicologic potential of otherwise nontoxic xenobiotics.7
Alkali metals (Group 1, formerly Group IA: Li, Na, K, Rb, Cs, Fr) and hydrogen (not an alkali metal on Earth) have a single outer valence electron and lose this electron easily to form compounds with a valence of 1+. The alkaline earth metals (Group 2, formerly Group IIA: Be, Mg, Ca, Sr, Ba, Ra) are located between the alkali and rare earth (Group 3, formerly Group IIIB), readily lose 2 electrons, and form cations with a 2+ charge. In their metallic form, members of both of these groups react violently with water to liberate strongly basic solutions, accounting for the word alkali in their group names (2Na0 + 2H2O → 2NaOH + H2).
The soluble ionic forms of sodium, potassium, or calcium, which are critical to survival, also produce life-threatening symptoms following excessive intake (Chap. 12). Xenobiotics interfere with the physiologic role of these key electrolytes. Li+ mimics K+ after entering neurons through K+ channels, and serves as an inadequate substrate for the repolarizing of Na+,K+-ATPase. Li+ thus interferes with cellular K+ homeostasis and alters neuronal repolarization, accounting for the neuroexcitability manifesting as tremor. Similarly, as noted previously, the molecular effects of Mg2+ and Ba2+ supplant those of Ca2+.
More commonly, the consequential toxicities ascribed to alkali or alkaline earth salts relate to the anionic component. In the case of NaOH or Ca(OH)2, it is the hydroxide anion, whereas with potassium cyanide (KCN) it is the cyanide (CN–) anion. When the chemical reactivity of an ionic compound (including its cellular toxicity) can be ascribed solely to one of the ions, any other ion in the compound is referred to as a spectator ion.
Unlike the alkali and alkaline earth metals, most other metallic elements are neither soluble nor reactive. This includes the transition metals (Groups 4-12; formerly Groups IB to VIIB, VIII, IB and IIB), a large group that contains several ubiquitous metals such as iron (Fe) and copper (Cu). These elements, in their metallic form, are widely used in both industrial and household applications because of their high tensile strength, density, and melting point, which is partly a result of their ability to delocalize the electrons in the d orbital throughout the metallic lattice. Transition metals also form brightly colored salts that find widespread applications, including as pigments for paints or fireworks. The ionic forms of these elements are of greater toxicologic importance than their metallic/elemental forms. Chemically, transition elements form at least one ion with a partially filled subshell of d electrons. Because the transition metals have partially filled valence shells, they are capable of obtaining several, usually positive, oxidation states. This important mechanism explains the role of transition metals in oxidation/reduction (redox) reactions, generally as electron acceptors (see Reduction-Oxidation below). This reactivity is used by organisms in various physiologic, catalytic, and coordination roles, such as at the active sites of enzymes and in hemoglobin. As expected, the substantial reactivity of these transition metal elements is associated with cellular injury caused by several mechanisms, including the generation of reactive oxygen species (Fig. 10–2). Manganese ion, as an example, is implicated in free radical damage of the basal ganglia, causing parkinsonism.
Heavy metal is often loosely used to describe all metals of toxicologic significance, but in reality the term should be reserved to describe only those metals in the lower period of the periodic table, particularly those with atomic masses greater than 200 Da. The chemical properties and toxicologic predilection of these elements vary, but a unifying toxicologic mechanism is electrophilic interference with nucleophilic sulfhydryl-containing enzymes. Some of the heavy metals also participate in the generation of free radicals through Fenton chemistry (Fig. 10–2). The likely determinant of the specific toxicologic effects produced by each metal is the tropism for various physiologic systems, enzymes, or microenvironments; thus, lipophilicity, water solubility, ionic size, and other physicochemical parameters are undoubtedly critical. Also, because the chemistry of metals varies dramatically based on the chemical form (ie, organic, inorganic, or elemental), as well as the charge on the metal ion, prediction of the clinical effects of a particular metal is often difficult.
Elemental mercury (Hg0) is unique in that it is the only metal that exists in liquid form at room temperatures. As such, it is capable of creating semisolid solutions, or amalgams, with other metals. Although relatively innocuous if ingested as a liquid, elemental mercury is readily volatilized (as a result of its high vapor pressure), transforming it into a significant pulmonary mucosal irritant upon inhalation. Pulmonary exposure increases its systemic bioavailability. Elemental mercury undergoes biotransformation in the erythrocyte and brain to the mercuric (Hg2+) form, which has a high affinity for sulfhydryl-containing molecules, such as proteins. This causes a depletion of glutathione in organs such as the kidney, and also initiates lipid peroxidation. The mercurous form (Hg+) is considerably less toxic than the mercuric (Hg2+) form, perhaps because of its reduced water solubility, which limits absorption. Organic mercurial compounds, such as methylmercury and dimethylmercury, are formed environmentally by anaerobic bacteria containing the methylating compound methylcobalamin, a vitamin B12 analog (Chap. 95).
Metallic thallium (Tl0) is used in the production of electronic equipment, and is itself minimally toxic. Thallium ions, however, have physicochemical properties that closely mimic potassium ions, allowing them to participate in and often alter the myriad physiologic activities related to potassium. This property is exploited during a thallium-stress test to assess for myocardial ischemia or infarction; because ischemic myocardial cells lack adequate energy for normal Na+,K+-ATPase function, they cannot exchange sodium for potassium (or radioactive thallium administered during a stress test). This produces a “cold spot” in the ischemic areas on cardiac scintigraphy (Chap. 99).
Lead is not abundant in the Earth’s crust (0.002%), but mining and industrial use make it a significant toxicologic concern. Lead exposure occurs during the smelting process or from one of its diverse commercial applications. Most of the useful lead compounds are inorganic plumbous (Pb2+) salts, but plumbic (Pb4+) compounds are also used. The Pb2+ compounds are typically ionizable, releasing Pb2+ when dissolved in a solvent, such as water. Lead ions are absorbed in place of Ca2+ ions by the gastrointestinal tract and replace Ca2+ in certain physiologic processes. This mechanism is implicated in the neurotoxic effect of lead ions. Lead compounds tend to be covalent compounds that do not ionize in water. Some Pb4+ compounds are oxidants. Although elemental lead is not itself toxic, it rapidly develops a coating of toxic lead oxide or lead carbonate on exposure to air or water (Chap. 93).
Although the metalloids (B, Si, Ge, As, Sb, Te, At) share many physical properties with metals, they differ in their propensity to form compounds with both metals and the nonmetals carbon, nitrogen, or oxygen. Metalloids are either oxidized or reduced in chemical reactions.
Toxicologically important inorganic arsenic compounds exist in either the pentavalent arsenite (As5+) form or the trivalent arsenate (As3+) form. The reduced water solubility of arsenate compounds (such as arsenic pentoxide) accounts for its limited clinical toxicity when compared to trivalent arsenic trioxide. The trivalent form of arsenic is primarily a nucleophilic toxin, binding sulfhydryl groups and interfering with enzymatic function (Chaps. 11 and 86).
The nonmetals (C, N, P, O, S, Se, halogens) are highly electronegative and are toxic in either their compounded or their elemental form. The nonmetals with large electronegativity, such as O2 or Cl2, generally oxidize other elements in chemical reactions. Those with lesser electronegativity, such as C, behave as reducing agents.
In their highly reactive elemental form, which is a covalent dimer of halogen atoms, the halogens (F, Cl, Br, I, At) carry the suffix ine (eg, Cl2, chlorine). Halogens require the addition of one electron to complete their valence shell, and are strong oxidizing agents. Because they are highly electronegative, they readily form halides (eg, Cl–, chloride) by abstracting electrons from less electronegative elements. Thus, the halogen ions, in their stable ionic form, generally carry a charge of –1. The halides, although much less reactive than their respective elemental forms, are reducing agents. The hydrogen halides (eg, HCl, hydrogen chloride) are gases under standard conditions, but they ionize when dissolved in aqueous solution to form hydrohalidic acids (eg, HCl, hydrochloric acid). All hydrogen halides except HF (hydrogen fluoride) ionize nearly completely in water to release H+ and are considered strong acids. Because of its small ionic radius, lack of charge dispersion, and the intense electronegativity of the fluorine atom, HF ionizes poorly and is a weak acid. This specific property of HF has important toxicologic implications (Chap. 104).
Group 18 (formerly Group VIIIA): Inert Gases. Inert gases (He, Ne, Ar, Kr, Xe, Rn), also known as noble gases, maintain completed valence shells and are thus unreactive except under extreme experimental conditions. Despite their lack of chemical reactivity, the inert gases are toxicologically important as simple asphyxiants, causing hypoxia if they displace ambient oxygen from a confined space (Chap. 121). During high-concentration exposure, inert gases produce anesthesia, and xenon is used as an anesthetic agent. Radon, although chemically unreactive, is radioactive, and prolonged exposure is associated with the development of lung cancer.
Electrons are not generally shared evenly between atoms when they form a compound unless the bond is between the same elements, as in Cl2. When different elements are involved, one will exert a larger attraction for the shared electrons. The degree to which an element draws the shared electron is determined by the electronegativity of the element (Fig. 10–3). The electronegativity of each element was catalogued by Linus Pauling and relates to the ionic radius, or the distance between the orbiting electron and the nucleus, and the shielding effects of the inner electrons. Electronegativity rises toward the right of the periodic table, corresponding with the expected charge obtained on an element when it forms a bond. Fluorine has the highest electronegativity of all elements, which explains many of the toxicologic properties of fluorine.
Several types of bonds exist between elements when they form compounds. When one element gains valence electrons and another loses them, the resulting elements are charged and attract one another in an ionic, or electrovalent, bond. An example is sodium chloride (NaCl), or table salt, in which the electronegativity difference between the elements is 1.9, or greater than the electronegativity of the sodium (Fig. 10–3). Thus, the chloride wrests control of the electrons in this bond. In solid form, ionic compounds exist in a crystalline lattice, but when put into solution, as in water or in blood (serum), the elements separate and form charged particles, or ions (Na+ and Cl–). The ions are stable in solution because their valence shells contain 8 electrons and are complete. The properties of ions differ from both the original atom from which the ion is derived and the noble gas with which it shares electronic structure.
It is important to recognize that when a mole of a salt, such as NaCl (molecular weight 58.45 g/mol), is put in aqueous solution, 2 moles of particles result. This is because NaCl is essentially fully ionized in water; that is, it produces one mole of Na+ (23 g/mol) and one mole of Cl– (35.5 g/mol). For salts that do not ionize completely, less than the intrinsic number of moles are released and the actual quantity liberated can be predicted based on the defined solubility of the compound, or the solubility product constant (Ksp). For ions that carry more than a single charge, the term equivalent is often used to denote the number of moles of other particles to which one mole of the substance will bind. Thus, one equivalent of calcium ions will typically bind 2 moles (or equivalents) of chloride ions (which are monovalent) because calcium ions are divalent. Alternatively stated, a 10% calcium chloride (CaCl2) aqueous solution contains approximately 1.4 mEq/mL or 0.7 mmol/mL of Ca2+.
Compounds formed by 2 elements of similar electronegativity have little ionic character because there is little impetus for separation of charge. Instead, these elements share pairs of valence electrons, a process known as covalence. The resultant molecule contains a covalent bond, which is typically very strong and generally requires a high-energy chemical reaction to disrupt it. There is wide variation in the extent to which the electrons are shared between the participants of a covalent bond, and the physicochemical and toxicologic properties of any particular molecule are in part determined by its nature. Sharing is rarely symmetric (as in oxygen {O2} or chlorine {Cl2}). When electrons are shared asymmetrically (localized to a greater degree around one of the component atoms), the bond is called polar. Importantly, however, the presence of polar bonds does not mean that the compound itself is polar. For example, methane contains a carbon atom that shares its valence electrons with 4 hydrogen atoms, in which there is a small charge separation between the elements (electronegativity {EN} difference = 0.40). Although each of the carbon hydrogen bonds is polar, however, the tetrahedral configuration of the molecule means that the asymmetries cancel each other out, resulting in no net polarity, rendering the compound nonpolar. The lack of polarity demonstrates that methane molecules have little affinity for other methane molecules and they are held together only by weak intermolecular bonds. This explains why methane is highly volatile and therefore exists as a gas under standard temperature and pressure.
Because the EN differences between hydrogen (EN = 2.20) and oxygen (EN = 3.44) are significant (EN difference = 1.24), the electrons in the hydrogen-oxygen bonds in water are drawn toward the oxygen atom, giving it a partial negative charge and the hydrogen a partial positive charge. Because H2O is angular, not linear or symmetric, water is a polar molecule. Water molecules are held together by hydrogen bonds, which are bonds between electropositive hydrogen atoms and the strongly electronegative oxygen atoms. Hydrogen bonds are stronger than other intermolecular bonds (eg, van der Waals forces; see later). Hydrogen bonds have sufficient energy to open many ionic bonds and solvate ions (ie, cause ionic compounds to go into solution). In this process, the polar ends of the water molecule surround the charged particles of the dissolved salt. As one corollary, nonpolar molecules are relatively insoluble in polar compounds such as water, as there is little similarity between the nonpolar methane and the polar water molecules. As a second corollary, salts cannot be solvated by nonpolar compounds, and thus a salt, such as sodium chloride, has almost no solubility in a nonpolar solvent such as carbon tetrachloride.
Alternatively, the stability and irreversibility of the bond between an organic phosphorus compound and the cholinesterase enzyme are a result of covalent phosphorylation of an amino acid at the active site of the enzyme. The resulting bond is essentially irreversible in the absence of another chemical reaction (Fig. 110–3).
Compounds often share multiple pairs of electrons. For example, the 2 carbon atoms in acetylene (HC≡CH) share 3 pairs of electrons between them, and each shares one pair with a hydrogen. Carbon and nitrogen share 3 pairs of electrons in forming cyanide (C≡N–), making this bond very stable and accounting for the large number of xenobiotics capable of liberating cyanide. Complex ions are covalently bonded groups of elements that behave as a single element. For example, hydroxide (OH–) and sulfate (SO42–) form sodium salts as if they were simply the ion of a single element (such as chloride).
Noncovalent bonds, such as hydrogen or ionic bonds, are important in the interaction between ligands and receptors as well as between ion channels and enzymes. These are low-energy bonds and easily broken. Van der Waals forces, also known as London dispersion forces, are intermolecular forces that arise from induced dipoles as a consequence of nonuniform distribution of the molecular electron cloud. These forces become stronger as the atom (or molecule) becomes larger because of the increased polarizability of the larger, more dispersed electron clouds. This accounts for the fact that under standard temperature and pressure, fluorine and chlorine are gases, whereas bromine is a liquid, and iodine is a solid.
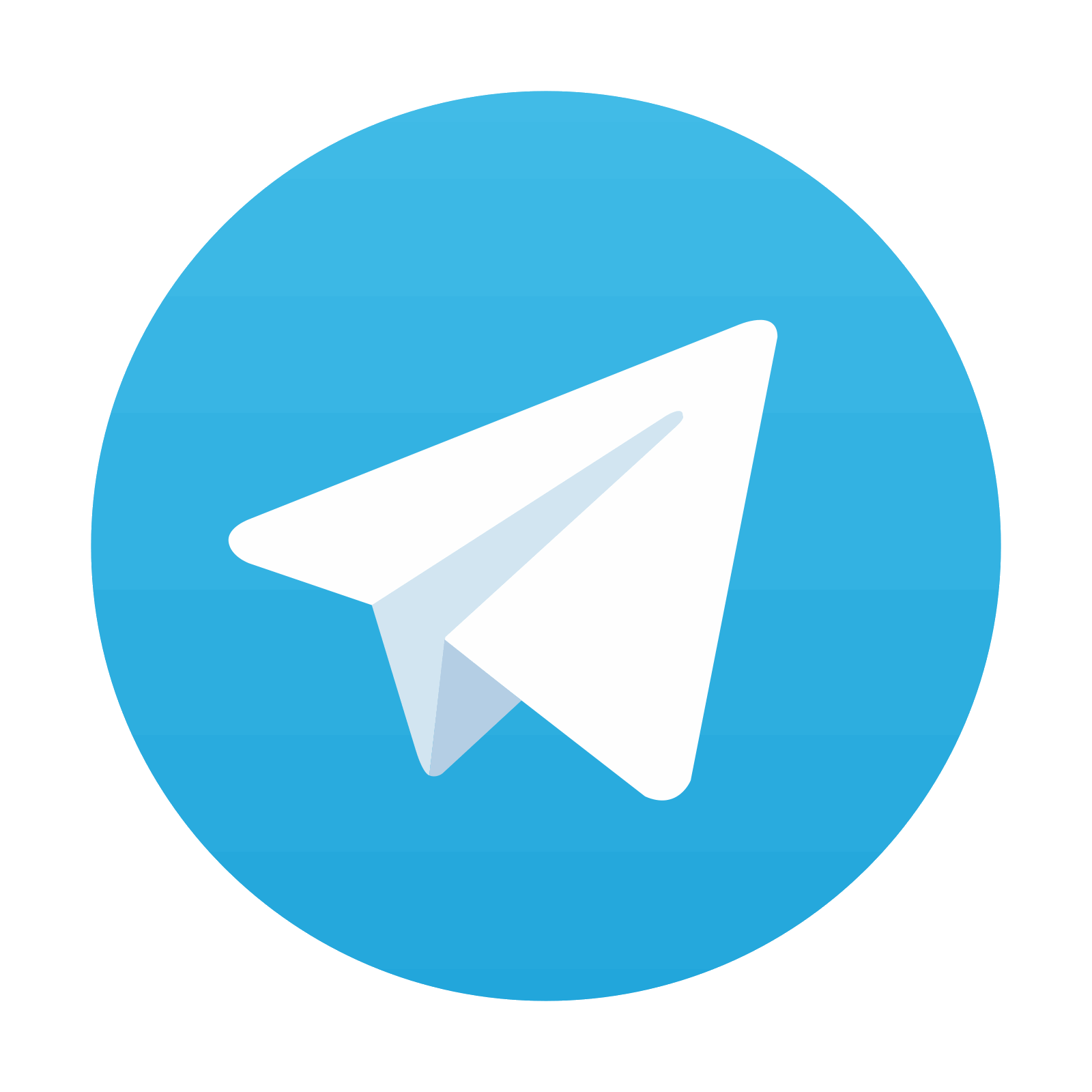
Stay updated, free articles. Join our Telegram channel
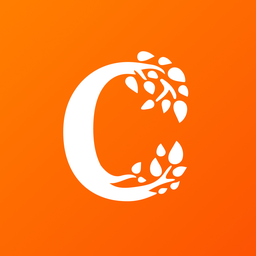
Full access? Get Clinical Tree
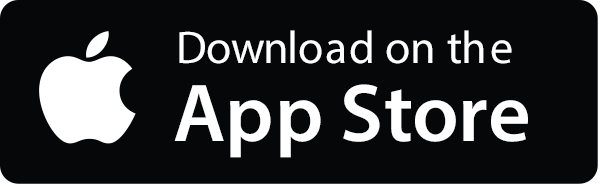
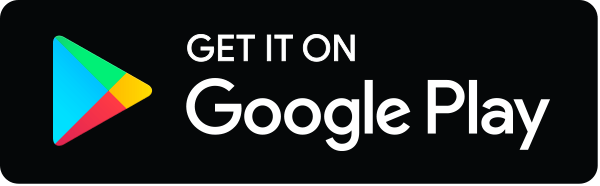