The kidneys lie in the paravertebral grooves at the level of the T12 to L3 vertebrae. The medial margin of each is concave, whereas the lateral margins are convex, giving the organ a bean-shaped appearance. In the adult, each kidney measures 10 to 12 cm in length, 5 to 7.5 cm in width, and 2.5 to 3.0 cm in thickness. In an adult man, each kidney weighs 125 to 170 g; in an adult woman, each kidney weighs 115 to 155 g.
On the medial surface of the kidney is the hilum, through which the renal artery, vein, renal pelvis, a nerve plexus, and lymphatics pass. On the convex surface, the kidney is surrounded by a fibrous capsule, which protects it, and a fatty capsule with a fibroareolar capsule called the renal fascia, which offers further protection and serves to anchor it in place.
The arterial supply begins with the renal arteries, which are direct branches of the aorta. On entering the hilum, the arteries subdivide into branches supplying the 5 major segments of each kidney: the apical pole, the anterosuperior segment, the anteroinferior segment, the posterior segment, and the inferior pole. These arteries subsequently divide within each segment to become lobar arteries. In turn, these vessels give rise to arcuate arteries that diverge into the sharply branching interlobular arteries, and then the afferent arterioles that directly supply the glomerular capillary tufts. The capillaries are then drained by the efferent arterioles, and peritubular capillaries, which also supply the vasa recta. Eventually, all are combined into the renal veins, which empty into the inferior vena cava.
The cut surface of the kidney reveals a pale outer rim and a dark inner region corresponding to the cortex and medulla, respectively. The cortex is 1 cm thick and surrounds the base of each medullary pyramid. The medulla consists of between 8 and 18 cone-shaped areas called medullary pyramids; the apex of each area forms a papilla containing the ends of the collecting ducts. Urine empties from each papilla into a calyx, and the calyces join together to form the renal pelvis. Urine is drained from the pelvis into the ureters and, subsequently, into the urinary bladder.
The kidneys serve 3 major functions: (1) homeostasis of fluids, acid–base balance, and electrolytes; (2) excretion of nitrogen, as urea, and other waste products; and (3) endocrine production such as 1,25-dihydroxy vitamin D, renin, and erythropoietin.
The kidneys maintain the constancy of the extracellular fluid by creating an ultrafiltrate of the plasma that is virtually free of cells and larger macromolecules, and then processing that filtrate, reclaiming what the body needs and letting the rest escape as urine. Every 24 hours, the adult’s glomeruli filter nearly 180 L of water (total body water is ~25–60 L) and 25,000 mEq of sodium (total body Na+ content is 1,200–2,800 mEq). Under normal circumstances, the kidneys regulate salt and water excretion, depending on intake and extrarenal losses. Only approximately 1% of the filtered water and 0.5% to 1% of the filtered Na+ are excreted.
Renal function begins with filtration of plasma at the glomerulus, a highly permeable capillary network connecting 2 arterioles in series. The relative constriction or dilation of these vessels normally controls the glomerular filtration rate (GFR). The glomerular capillaries are lined with endothelial cells, and encased by specialized epithelial cells called podocytes. The filtration barrier consists of the filtration slits, the connections between adjoining podocytes, and the glomerular basement membrane, the layers between the podocytes and endothelial cells. Under healthy circumstances, the filtration fraction—the proportion of renal plasma that is filtered into the Bowman space, the potential space surrounding the glomerular capillary tuft—is approximately 20%. Plasma water crosses the filter, along with electrolytes, small solutes such as glucose, amino acids, lactate, and urea; blood cells and most of the larger proteins, including albumin and globulins, do not typically cross the filter, although recent observations suggest that more plasma proteins, including albumin, than previously thought are filtered and reclaimed by the proximal tubule. The filtrate then enters the tubules, the serial segments of which have varying roles to metabolize, reabsorb, and secrete various solutes.
The proximal tubule performs the bulk of reabsorption, isotonically reclaiming 65% to 70% of the filtrate. It has 3 segments, called S1, S2, and S3. Distal to the proximal tubule is the hairpin turn of the thin limb of the loop of Henle, a segment important in maintaining the corticomedullary concentration gradient, which is important for urinary concentration and dilution. The thick ascending limb of the loop of Henle follows, which also influences concentration and dilution of the urine and plays an important role in the balance of Na+, Ca2+, and Mg2+. The distal convoluted tubules also reabsorb Na+ and Ca2+ and are joined by the connecting tubules to form the collecting ducts. These segments are relatively “tight” epithelia, allowing the establishment of gradients of solute concentration from blood to urine or vice versa. These distal segments are where the fine-tuning of excretion and reclamation of water, urea, K+, and H+ occurs.
Given the importance of sodium balance to maintenance of cardiac preload and cardiac output, it is reasonable to consider sodium reabsorption, which occurs to an extent in every segment, as the kidney’s most important role. Sodium reabsorption in the proximal tubule occurs both via both paracellular transport and transcellular transport. The former occurs in response to hydrostatic and oncotic pressures between the peritubular capillaries and the tubule, while the latter is mediated by a Na+–H+ exchanger stimulated by angiotensin II. In the distal tubule, hormones such as aldosterone, and a variety of kinases, control sodium transport.
Control of water balance includes urinary dilution or urinary concentration depending on the clinical circumstance. When dilution is appropriate, the ascending limb of the loop of Henle absorbs solute without water. This action produces a dilute tubular fluid and makes the medullary interstitium hypertonic. Suppression of the antidiuretic hormone (ADH; or vasopressin) leads to disappearance of water pores called aquaporins from the collecting duct; electrolyte-free water is then excreted. When urinary concentration is appropriate, ADH stimulates the appearance of aquaporins, allowing water to move from the tubule lumen into the interstitium, to minimize loss of electrolyte-free water.
The kidneys also regulate the balance of K+ and H+ (which are secreted by the distal nephron under the influence of aldosterone), and Ca2+ and phosphate via circulating parathyroid hormone, activated vitamin D, and fibroblast growth factor 23. The tubules also are capable of secretion of many solutes, including drugs such as diuretics, uric acid, and other organic anions.
Xenobiotics affect kidney function in various ways and, conversely, renal disease influences their pharmacokinetics, which sometimes leads to dangerous overdosing and potential toxicity. In this case, kidney failure, whether acute or chronic, reduces the clearance of xenobiotics eliminated by the kidneys. The ideal dosing of drugs such as gabapentin, digoxin, baclofen, and vancomycin must be reduced several-fold in patients with advanced kidney failure compared to those with intact kidney function. Some xenobiotics are potentially toxic in patients with kidney impairment while being relatively benign in patients with normal GFR. This is the case for gadolinium-based contrast used for magnetic resonance imaging, which carries the risk of nephrogenic systemic fibrosis.55
Hemodialysis or peritoneal dialysis further complicates pharmacokinetics and drug dosing; in particular, the elimination of many antimicrobials are severely altered in critically ill patients with kidney failure, whether acute or chronic. In this setting, the risk of underdosing with subsequent therapeutic failure and breakthrough resistance acquired by microorganisms occasionally surpasses that of drug accumulation. A notable example is fluconazole: the required dosage in patients undergoing continuous renal replacement therapy even surpasses the drug requirement in patients with intact kidney function.58 For those drugs of which a substantial fraction is removed by hemodialysis, drug re-dosing after dialysis is often recommended. The use of therapeutic guides is encouraged to ensure proper drug prescription in patients with a history of kidney disease.16
Many xenobiotics cause or aggravate renal dysfunction. The kidneys are particularly susceptible to toxic injury for 4 reasons: (1) they receive 20% to 25% of cardiac output, yet make up less than 1% of total body mass (~300 grams in a 70 kg man), implying a relatively large exposure to circulating xenobiotics; (2) they are metabolically active, and thus vulnerable to xenobiotics that disrupt metabolism or are activated by metabolism, such as acetaminophen (APAP); (3) they remove water from the glomerular filtrate, which increases the tubular concentration of xenobiotics; and (4) the glomeruli and interstitium are susceptible to attack by the immune system. Many factors, such as renal perfusion, affect an individual’s reaction to a particular nephrotoxin.3 Clinicians should be aware of these factors so as to minimize the adverse effect of a toxic exposure.
Xenobiotics can affect any part of the nephron (Fig. 27–1), although not every type of toxic renal exposure will result in loss of GFR. The following will be described: (1) acute kidney injury, (2) chronic kidney disease, and (3) functional kidney disorders.
Acute kidney injury (AKI; formerly called “acute renal failure”) relates to an abrupt decline in renal function that impairs the capacity of the kidney to maintain metabolic balance. Several definitions of AKI now exist, although they will likely be revised with the advent of newer and more specific biomarkers of kidney injury. The Kidney Disease: Improving Global Outcomes (KDIGO) clinical practice guidelines introduced staging criteria for AKI, based on prior work from the Acute Dialysis Quality Initiative (ADQI) and the Acute Kidney Injury Network (AKIN) (Table 27–1). The 3 traditional, main categories of AKI are prerenal, postrenal, and intrinsic AKI.
Stage 1 | Creatinine of 1.5–1.9 times baseline OR ≥0.3 mg/dL (≥26.5 μmol/L) increase in the serum creatinine OR Urine output <0.5 mL/kg/h for 6–12 hours |
Stage 2 | Creatinine of 2.0–2.9 times baseline increase in the serum creatinine OR Urine output <0.5 mL/kg/h for ≥12 hours |
Stage 3 | Creatinine of 3.0 times baseline increase in the serum creatinine OR Increase in serum creatinine to ≥4.0 mg/dL (≥353.6 μmol/L) OR Urine output of <0.3 mL/kg/h for ≥ 24 hours OR Anuria for ≥12 hours OR Initiation of renal replacement therapy OR In patients <18 years, decrease in estimated GFR to <35 mL/min per 1.73 m2 |
Prerenal AKI implies impaired renal perfusion, which occurs with volume depletion, systemic vasodilation, heart failure, or preglomerular vasoconstriction. Renal hypoperfusion initiates a sequence of events leading to renal salt and water reabsorption.3 Renin is released, causing production of angiotensin II, which enhances proximal tubular sodium reabsorption and stimulates adrenal aldosterone release, thus increasing distal sodium reabsorption. Therefore, prerenal AKI is usually accompanied by low urinary sodium excretion. Release of ADH increases water retention, manifested by a high urine osmolality. Histologically, the kidney appears normal.
Any toxic exposure that compromises renal perfusion potentially contributes to prerenal AKI, including bleeding (eg, from overdose of anticoagulants), volume depletion (diuretics, cathartics, or emetics), cardiac dysfunction (β-adrenergic antagonists), or hypotension from any cause.53 Nonsteroidal antiinflammatory drugs (NSAIDs) lower the filtration rate by inhibiting production of vasodilatory prostaglandins in the afferent arteriole. Angiotensin-converting enzyme inhibitors (ACEIs) and angiotensin receptor blockers (ARBs) reduce the vasoconstrictive effect of angiotensin II on the efferent arteriole and can both cause prerenal AKI, especially in the setting of volume contraction. Finally, cardiotoxins, such as doxorubicin, cause severe heart failure (Fig. 27–1). Calcineurin inhibitors (cyclosporine, tacrolimus) also cause prerenal vasoconstriction by their effect on both afferent and efferent arterioles, possibly induced by endothelin. Calcineurin nephrotoxicity is usually dose-dependent and especially occurs when trough concentrations remain supratherapeutic for an extended period of time. Nephrotoxicity is often reversible after temporary discontinuation or decrease in the calcineurin dose if identified relatively quickly.
Prerenal AKI is also caused by the hepatorenal syndrome, which is characterized by progressive renal hypoperfusion in the context of severe acute or chronic liver failure. In this setting, marked constriction of the renal arterial vasculature coexists with profound systemic and splanchnic hypotension, caused by several circulating mediators, including angiotensin, norepinephrine, vasopressin, endothelin, and isoprostane F2.50 That the cause of AKI is extrarenal is best illustrated by the fact that the histology of a kidney from a patient with hepatorenal syndrome is completely normal. The prognosis of the hepatorenal syndrome is entirely dependent on the recovery of liver function.
Finally, the entity known as the abdominal compartment syndrome is a potential cause of renal hypoperfusion.9 Abdominal compartment syndrome is usually defined as new organ dysfunction induced by intraabdominal hypertension and occurs when the intraabdominal pressure exceeds 20 mm Hg, especially if sustained. The abdominal compartment syndrome is caused by several entities, but usually requires some abdominal event, especially with concomitant aggressive fluid resuscitation. Surgical decompression is life-saving in this context.
Renal AKI implies intrinsic damage to the renal parenchyma, which is divided into vascular, glomerular, and tubulointerstitial etiologies.
Vascular etiologies of AKI include vasculitis, malignant hypertension, atheroemboli, scleroderma, and hemolytic-uremic syndrome or thrombotic thrombocytopenic purpura (HUS/TTP), the latter of which is sometimes associated with use of certain xenobiotics (Table 27–2).
Glomerular diseases infrequently cause acute AKI, but more commonly either a chronic or subacute decline in kidney function. Glomerular lesions either present with the nephrotic or nephritic syndrome. A nephritic pattern is associated with histological inflammation, an active urine sediment with proteinuria and hematuria, and impaired kidney function. Hypertension is common. The nephrotic syndrome is characterized by massive proteinuria (>3.5 g/day), hypoalbuminemia, hyperlipidemia, and pitting pedal edema that usually prompts the patient to seek medical attention. Although the relationships between these findings are not completely understood, the underlying event is injury to the glomerular barrier that normally prevents macromolecules from passing from the capillary lumen into the urinary space.
Xenobiotics induce nephrotic syndrome (Table 27–3) in 2 ways. First, they release antigens into the blood, which leads to antigen–antibody complex formation after the immune response is elicited. These complexes such as those of gold salts subsequently deposit in the glomerular basement membrane, thereby changing its properties19 (Fig. 27–2). Second, they upset the immunoregulatory balance.
Anabolic steroids |
Antimicrobials (cefixime, rifampicin) |
Captopril |
Chemotherapeutics (bevacizumab, sunitinib, sorafenib) |
Drugs of abuse (heroin, cocaine) |
Insect venom |
Interferon α |
Metals (Au, Hg, Li) |
Nonsteroidal antiinflammatory drugs |
Pamidronate |
Penicillamine |
Probenecid |
Sirolimus |
Kidney biopsy will permit identification of the characteristic pathologic pattern, which includes but is not limited to minimal glomerular change disease, membranous glomerulopathy, or focal segmental glomerulosclerosis. Hypoalbuminemia usually is worse than urinary excretion of albumin would suggest, as a result of tubular metabolism of filtered protein. The tubules also retain sodium, causing expansion of the extracellular space and edema. The glomerular lesion will progress to end-stage renal disease (ESRD) if the pathologic process continues.
The major causes of acute tubulointerstitial diseases include acute tubular necrosis (ATN), acute interstitial nephritis (AIN), and tumor lysis syndrome. Although there is controversy about how a tubular lesion leads to glomerular shutdown, it is generally felt that tubular obstruction, back-leak of filtrate across injured epithelium, renal hypoperfusion, and decreased glomerular filtering surface combine to impair glomerular filtration.5,60 Additionally, filtration pressure is diminished by neutrophil infiltration into the interstitium and vasa recta. Evidence also suggests that prolonged medullary ischemia, perhaps caused by an imbalance in the production of vasoconstrictors such as endothelin and vasodilators such as nitric oxide, is important in prolonging the renal dysfunction after the tubular injury develops.32
Acute tubular necrosis is the most common cause of AKI in hospitalized patients (Table 27–4). Acute tubular necrosis is manifested pathologically by patchy necrosis of the tubular epithelium and occlusion of the lumen by casts and cellular debris (Fig. 27–3). Clinically, ATN presents as a rapid deterioration of kidney function. Muddy brown casts or renal tubular cells are typically seen in the urinary sediment, but hematuria and leukocyturia are unusual. Disorders of metabolic balance, such as hyperkalemia and metabolic acidosis, are also common. The abrupt fall in GFR usually leads to positive sodium and water balance.39
Acetaminophen |
Aluminum phosphide |
Antibacterials (aminoglycosides, ciprofloxacin, levofloxacin, colistin) |
Antifungals (amphotericin B, pentamidine) |
Antivirals (acyclovir, foscarnet, ritonavir, tenofovir, cidofovir, adefovir) |
Chemotherapeutics (cisplatin, ifosfamide, mithramycin, streptozotocin) |
Deferoxamine |
Etidronate |
Grass carp gallbladder |
Halothane |
Metals (As, Bi, Cr, Hg, U) |
Mushrooms (Amanita {especially A. smithiana}), Cortinarius spp) |
Non-protein colloids (mannitol, hydroxyethyl starches, dextran) |
Paraquat, diquat |
Radiocontrast agents (iodinated contrast, gadolinium) |
Acute tubular necrosis occurs following an ischemic or toxic injury.5,46,60 Direct toxicity accounts for approximately 35% of all cases of ATN. Xenobiotics affect different segments of the renal tubules; for example, uranium and other heavy metals react with the proximal tubule and amphotericin the distal tubule (Fig. 27–1).49 Certain xenobiotics cause a sudden or progressive decrease in GFR with concomitant prominent tubular wasting for electrolytes, though mechanisms of injury vary; this is the case of ifosfamide,20 amphotericin B,49 aminoglycosides,48 pentamidine,37 and cisplatin.11 Poisoning also leads to ischemic tubular necrosis if hypotension or cardiac failure causes prolonged ischemia of nephron segments (proximal straight tubule and inner medullary collecting duct) that are particularly vulnerable to hypoxia. Patients who receive significant amounts of certain colloids (specifically mannitol, dextran, or hydroxyethyl starch)13 occasionally develop AKI, characterized by tubular vacuolization or “osmotic nephrosis.” Iodinated contrast causes AKI by mediating medullary vasoconstriction and inducing reactive oxygen species in tubules, despite recent studies questioning the existence of this phenomenon. Whatever the clinical pattern of rapidly declining renal function, all forms of ATN usually present with oliguria, aside from toxicity to aminoglycosides that can present as non-oliguric ATN.7
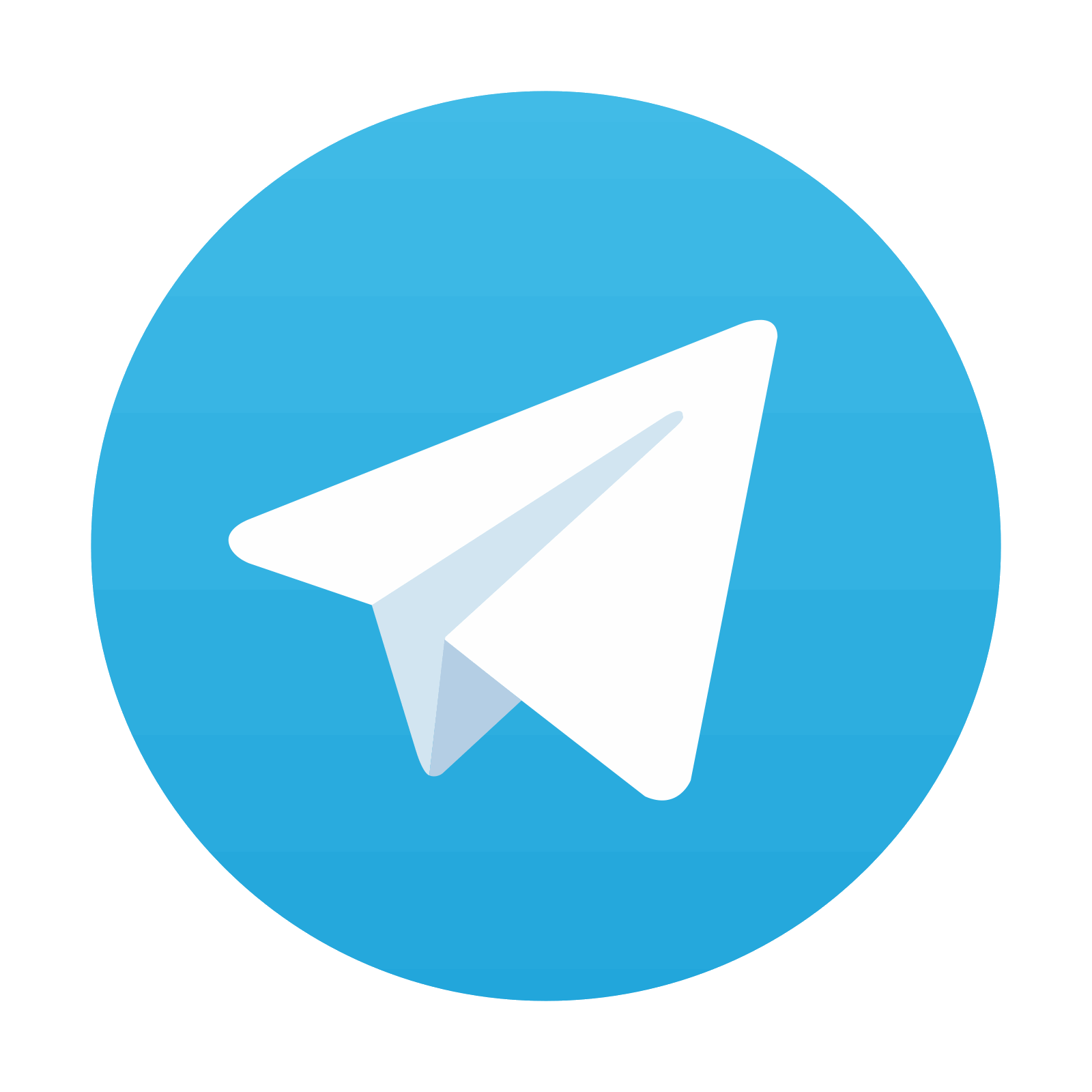
Stay updated, free articles. Join our Telegram channel
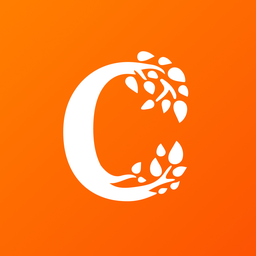
Full access? Get Clinical Tree
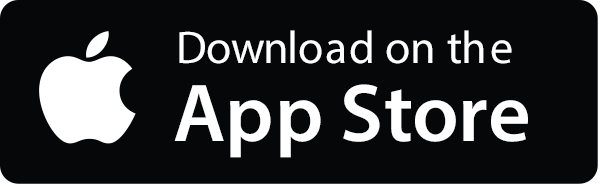
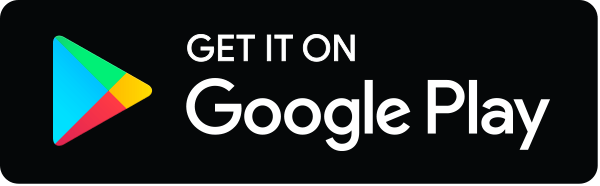