© Springer-Verlag Berlin Heidelberg 2015
Hans-Georg Schaible (ed.)Pain ControlHandbook of Experimental Pharmacology22710.1007/978-3-662-46450-2_6Nitric Oxide-Mediated Pain Processing in the Spinal Cord
(1)
Institut für Pharmakologie und Toxikologie, Universität Witten/Herdecke, ZBAF, Stockumer Str. 10, 58453 Witten, Germany
Abstract
A large body of evidence indicates that nitric oxide (NO) plays an important role in the processing of persistent inflammatory and neuropathic pain in the spinal cord. Several animal studies revealed that inhibition or knockout of NO synthesis ameliorates persistent pain. However, spinal delivery of NO donors caused dual pronociceptive and antinociceptive effects, pointing to multiple downstream signaling mechanisms of NO. This review summarizes the localization and function of NO-dependent signaling mechanisms in the spinal cord, taking account of the recent progress made in this field.
Keywords
PainNociceptionDorsal root gangliaSpinal cordNitric oxidecGMPAbbreviations
cGKI
cGMP-dependent protein kinase I (synonym PKG-1, protein kinase G-1)
cGMP
3′, 5′-cyclic guanosine monophosphate
CNG
Cyclic-nucleotide gated
DRG
Dorsal root ganglion
GC-A
Particulate guanylyl cyclase A (synonym NPR-A, natriuretic peptide receptor A)
GC-B
Particulate guanylyl cyclase B (synonym NPR-B, natriuretic peptide receptor B)
HCN
Hyperpolarization activated and cyclic-nucleotide gated
NO
Nitric oxide
NO-GC
NO-sensitive guanylyl cyclase (synonym sGC, soluble guanylyl cyclase)
NOS
NO synthase
PDE
Phosphodiesterase
1 Expression of NO Synthases in the Spinal Cord and in Dorsal Root Ganglia
Nitric oxide (NO) serves as a key biological signal in the regulation of many physiological and pathophysiological functions (Francis et al. 2010). It is a small gaseous molecule with a half-life of several seconds that readily permeates cell membranes. As NO cannot be stored in vesicles and secreted in a controlled fashion, its functions are primarily regulated by the expression and activity of NO synthases (NOSs) that produce NO and l-citrulline from the precursor l-arginine. Three different NOS isoforms have been identified that are encoded by three distinct genes. According to their primary origins or properties, NOS isoforms are referred to as neuronal NOS (nNOS or NOS-1), inducible NOS (iNOS or NOS-2), and endothelial NOS (eNOS or NOS-3). Both nNOS and eNOS are expressed constitutively, exhibit low basal activity, and are stimulated by Ca2+ influx and Ca2+/calmodulin binding. iNOS is induced in response to inflammatory stimuli, and its activity does not depend on intracellular Ca2+. The activities of NOS enzymes are regulated by several mechanisms, including phosphorylation, nitrosylation, interaction with other proteins, cofactor/substrate availability, and changes in transcription (Bian et al. 2006; Francis et al. 2010).
A large body of evidence indicates that nNOS is a major source of NO during pain processing in the dorsal horn of the spinal cord. Under basic conditions, nNOS is constitutively expressed in some neurons (5–18 % of total neurons) in laminae I–III (Valtschanoff et al. 1992; Dun et al. 1993; Spike et al. 1993; Zhang et al. 1993; Herdegen et al. 1994; Laing et al. 1994; Saito et al. 1994; Bernardi et al. 1995; Ruscheweyh et al. 2006; Sardella et al. 2011; Gassner et al. 2013). Double-labeling immunostaining experiments detected nNOS in a subpopulation of GABAergic inhibitory neurons which innervate giant projection neurons in lamina I (Puskar et al. 2001) and only sparsely overlap with other subpopulations of inhibitory neurons positive for neuropeptide Y, galanin, and parvalbumin (Laing et al. 1994; Tiong et al. 2011; Polgar et al. 2013). nNOS is also expressed at a relatively low level by excitatory interneurons positive for protein kinase Cγ in laminae II and III of the spinal cord (Hughes et al. 2008; Sardella et al. 2011) and in the somata of a few (<5 %) dorsal root ganglion (DRG) neurons (Aimi et al. 1991; Valtschanoff et al. 1992; Zhang et al. 1993; Henrich et al. 2002; Ruscheweyh et al. 2006).
With regard to the pain-relevant functions of NO, it is important to note that nNOS expression in the dorsal horn and in DRGs is considerably upregulated during the processing of persistent pain. Several animal studies demonstrated that the number of nNOS-immunoreactive dorsal horn neurons and the optical nNOS density in the dorsal horn are increased during inflammatory pain evoked by injection of proinflammatory agents such as formalin, zymosan, or complete Freund’s adjuvant into a hindpaw (Herdegen et al. 1994; Yonehara et al. 1997; Maihofner et al. 2000; Chu et al. 2005). In contrast, during neuropathic pain in response to peripheral nerve injury, nNOS expression was primarily upregulated in DRG neurons, leading to an increased number of nNOS-positive DRG neurons and enhanced nNOS immunoreactivity in their central terminals in the dorsal horn of the spinal cord (Zhang et al. 1993; Luo et al. 1999; Guan et al. 2007; Martucci et al. 2008). Hence, nNOS seems to play a particular role in the processing of persistent inflammatory and neuropathic pain in the spinal cord and is expressed in different neuronal populations.
Unlike nNOS, iNOS is, if at all, only weakly expressed in the dorsal horn and in DRGs under basic conditions (Wu et al. 1998; Maihofner et al. 2000; Henrich et al. 2002; Keilhoff et al. 2002; Chu et al. 2005; Ruscheweyh et al. 2006; Tang et al. 2007; Martucci et al. 2008). Data about iNOS induction in response to painful stimuli are not consistent. Whereas some studies reported iNOS induction in the spinal cord during the processing of inflammatory and/or neuropathic pain (Guhring et al. 2000; Tao et al. 2003; Martucci et al. 2008; Hervera et al. 2012), other studies reported that iNOS was not induced by painful stimuli (Keilhoff et al. 2002; Chu et al. 2005; De Alba et al. 2006; Guan et al. 2007). Moreover, the cellular distribution of iNOS in the spinal cord remains unclear. Finally, eNOS is constitutively expressed in vascular structures of the dorsal horn and DRGs (Keilhoff et al. 2002; Chu et al. 2005; Ruscheweyh et al. 2006), and its expression seems not to be regulated during pain processing (Keilhoff et al. 2002; Chu et al. 2005; Guan et al. 2007).
2 Pro- and Antinociceptive Functions of NO
The first evidence for a functional contribution of NO to pain processing was discovered in studies using NOS inhibitors such as l-NAME and l-NMMA, which inhibit all three NOS isoforms in a nonspecific manner. These early studies revealed that intrathecal (i.t.) administration of NOS inhibitors effectively ameliorated the pain behavior in various rodent models of inflammatory and neuropathic pain (for review, see Meller and Gebhart 1993; Luo and Cizkova 2000). Experiments with more selective NOS isoform inhibitors point to an important role of nNOS in the development and maintenance of inflammatory and neuropathic pain (Tao et al. 2004; Chu et al. 2005; Guan et al. 2007; Dableh and Henry 2011) and to a contribution of iNOS to the processing of inflammatory pain (Guhring et al. 2000; Tao et al. 2003).
In addition to NOS inhibitors, NOS isoform-specific knockout mice were used to investigate the pain-relevant functions of NO. Many of these studies revealed that persistent pain behaviors were moderately reduced in nNOS and iNOS but not in eNOS knockout mice. However, the interpretation of the pain behavior in mice lacking a NOS isoform is complicated by the fact that the expression of other NOS isoforms may be compensatory upregulated (Tao et al. 2003, 2004; Boettger et al. 2007; Hervera et al. 2010). Moreover, in the widely used nNOS knockout mouse line with targeted deletion of exon 2, alternatively spliced nNOS variants that are functionally active (such as nNOSβ) are still present in distinct tissues (Eliasson et al. 1997). These obstacles may account for the relatively modest pain phenotypes observed in mice lacking nNOS and/or iNOS (Guhring et al. 2000; Tao et al. 2003, 2004; Chu et al. 2005; Boettger et al. 2007; Guan et al. 2007; Hervera et al. 2010; Kuboyama et al. 2011; Keilhoff et al. 2013).
Because inhibition or knockout of NO synthesis ameliorated persistent pain, NO donors were expected to have mainly pronociceptive effects. Indeed, it has been observed that intrathecally administered NO donors may induce or increase hyperalgesia (Kitto et al. 1992; Meller et al. 1992; Machelska et al. 1998; Ferreira et al. 1999; Lin et al. 1999). However, other studies revealed that NO may also have antinociceptive properties within the spinal cord (Luo and Cizkova 2000). For example, i.t. administration of the NO precursor, l-arginine, reduced the activity of dorsal horn neurons and increased the mechanical threshold for tail withdrawal (Haley et al. 1992; Zhuo et al. 1993). Several studies suggested that the concentration of NO may be an important determinant to explain these dual pro- and antinociceptive effects. For example, neuropathic and postoperative pain behavior of rats was inhibited by administration of low doses of an NO donor, while it was further increased by high doses (Sousa and Prado 2001; Kina et al. 2005). Furthermore, dose-dependent dual NO effects have also been observed in humans: NO administration via a transdermal nitroglycerin patch reduced pain due to shoulder or elbow injury at low NO doses (Berrazueta et al. 1996; Paoloni et al. 2003) and enhanced opioid analgesia (Lauretti et al. 1999a, b). Conversely, high doses of transdermal nitroglycerin patches or ointment induced hyperalgesia (Lauretti et al. 1999a; Cadiou et al. 2007). Altogether, there is considerable evidence that inhibition of NO production in the spinal cord ameliorates persistent inflammatory and neuropathic pain. In contrast, delivery of NO donors may exert both pro- and antinociceptive effects, pointing to different downstream signaling pathways of NO action (Schmidtko et al. 2009).
3 Downstream Mechanisms of NO-Mediated Pain Processing
3.1 Activation of NO-GC
At nanomolar levels, NO binds to a prosthetic heme of NO-sensitive guanylyl cyclase (NO-GC; also referred to as soluble guanylyl cyclase, sGC) and causes the conversion of GTP to cGMP (Francis et al. 2010). NO-GC is a heterodimer consisting of two different subunits termed α and β. Two catalytically active isoforms have been identified (α1β1 and α2β1) in which the β1 subunit acts as the dimerizing partner for the α1 or α2 subunit (Friebe et al. 2007). There is considerable evidence that NO-GC is a major NO target during pain processing. Mice deficient for the β1 subunit (GC-KO mice), which are completely devoid of NO-GC activity, failed to develop pain sensitization induced by intrathecal administration of NO donors. GC-KO mice also demonstrated considerably reduced pain behaviors in inflammatory and neuropathic pain models, whereas the immediate responses to acute nociceptive stimuli were normal (Schmidtko et al. 2008a). The important role of NO-GC for persistent pain processing in the spinal cord is further supported by antinociceptive effects of the NO-GC inhibitor ODQ after intrathecal injection in models of inflammatory and neuropathic pain (Ferreira et al. 1999; Kawamata and Omote 1999; Tao and Johns 2002; Song et al. 2006). Moreover, similar to NO donors, both pronociceptive and antinociceptive effects were observed after i.t. administration of cGMP analogs (Garry et al. 1994; Iwamoto and Marion 1994; Ferreira et al. 1999; Song et al. 2006), and again the administered dose seems to be a determinant for this dual effect (Tegeder et al. 2002, 2004; Schmidtko et al. 2008b). Dual effects of NO and cGMP were also observed in electrophysiological studies with spinal cord slices, in which superfusion with both NO donors and cGMP analogs inhibited ~50 % but activated ~30 % of dorsal horn neurons (Pehl and Schmid 1997).
The most likely reason for the dual effects of NO donors and cGMP analogs is the presence of different pronociceptive and antinociceptive NO/cGMP downstream signaling mechanisms. Unlike the membrane-permeable gas NO, cGMP mainly acts in intracellular compartments at its site of production. Interestingly, the expression pattern of NO-GC in the spinal cord and in DRGs suggests that NO-mediated cGMP production can modulate pain processing at different sites. In the spinal cord, NO-GC immunoreactivity is enriched in inhibitory interneurons in laminae II and III, i.e., in the area of highest nNOS expression (see above). NO-GC is also expressed in neurokinin 1 (NK1) receptor-positive projection neurons in lamina I (Ding and Weinberg 2006; Ruscheweyh et al. 2006; Schmidtko et al. 2008a). These cells not only contribute to the ascending conduction of pain but are also essential for NO-dependent long-term potentiation (LTP) at the first synapse in pain pathways (Mantyh and Hunt 2004; Ikeda et al. 2006). In DRGs, however, specific NO-GC immunoreactivity was unexpectedly not detected in neurons. Instead thereof, NO-GC protein seems to be present only in satellite cells and vascular cells (Schmidtko et al. 2008a). This finding is supported by observations that axotomy of the sciatic nerve or incubation of DRG sections with an NO donor initiated cGMP production selectively in non-neuronal DRG cells (Morris et al. 1992; Shi et al. 1998). Considering that peripheral nerve injury leads to nNOS upregulation in somata of DRG neurons (see above) and that satellite cells contain NO-GC, it is likely that NO acts as a paracrine messenger from DRG neurons to satellite cells, thereby possibly contributing to the satellite cell proliferation in response to peripheral nerve injury (Zhuang et al. 2005; Scholz and Woolf 2007; Zhang et al. 2007; Kawasaki et al. 2008). Importantly, the observation that NO-GC is not expressed in primary afferent neurons challenges an earlier hypothesis that NO might act as “retrograde” transmitter which is released by spinal cord neurons and stimulates cGMP production via NO-GC activation in primary afferent neurons (Meller and Gebhart 1993; Luo and Cizkova 2000). Instead thereof, NO seems to be primarily a transmitter that (1) is released from nNOS-positive DRG neurons and dorsal horn interneurons (and possibly from so far unidentified iNOS-positive cells) and (2) induces cGMP production in DRG satellite cells, in lamina I projection neurons, and in laminae II/III inhibitory interneurons (Schmidtko et al. 2009).
3.2 cGMP Signaling
The elucidation of downstream mechanisms of NO/cGMP signaling in the nociceptive system has been complicated by at least two facts: First, cGMP in general signals by various mechanisms including activation of cGMP-dependent protein kinase (cGK; also referred to as protein kinase G, PKG), activation of cyclic-nucleotide-gated (CNG) channels, modulation of hyperpolarization-activated and cyclic-nucleotide-gated (HCN) channels, and modulation of phosphodiesterases (PDEs) (Craven and Zagotta 2006; Feil and Kleppisch 2008). Recent data indicate that all these cGMP targets are present in the nociceptive system. Second, cGMP is produced not only by NO-GC but also in a NO-independent manner by particulate guanylyl cyclases in response to stimulation by natriuretic peptides. Seven particulate guanylyl cyclase isoforms activated by different ligands have been identified in rodents (Garbers et al. 2006), and particulate guanylyl cyclases A and B (GC-A and GC-B; also referred to as natriuretic peptide receptor A [NPR-A] and natriuretic peptide receptor B [NPR-B], respectively) have been detected in DRG neurons (Schmidt et al. 2007; Kishimoto et al. 2008; Schmidtko et al. 2008a; Zhang et al. 2010; Loo et al. 2012).
After the discovery of pain-relevant NO/cGMP signaling in the 1990s, it was initially thought that most effects of NO and cGMP are mediated by cGKI (Qian et al. 1996), corresponding to the functional NO/NO-GC/cGMP/cGKI signaling pathway that exists in many other tissues (Feil and Kleppisch 2008). More recent studies confirmed the important pain-relevant role of cGKI, but cGKI seems to be mainly activated by NO-independent mechanisms during pain processing (see below). Several immunohistochemical studies detected the α-isoform of cGKI in the majority of DRG neurons and their nerve terminals in the spinal cord and in some dorsal horn neurons (Qian et al. 1996; Tao et al. 2000; Sung et al. 2006; Schmidtko et al. 2008b; Luo et al. 2012; Lorenz et al. 2014). After peripheral nerve injury and inflammation, cGKIα is activated in DRG neurons (Sung et al. 2004, 2006; Lorenz et al. 2014), and its expression increases in the spinal cord (Tao et al. 2000; Tegeder et al. 2002; Schmidtko et al. 2003). The essential contribution of cGKIα to persistent pain processing is reflected by the reduced inflammatory and/or neuropathic pain behavior in global or nociceptor-specific cGKI mutants (Tegeder et al. 2004; Luo et al. 2012; Lorenz et al. 2014) and by profound antinociceptive effects of intrathecally administered cGKI inhibitors (Tao et al. 2000; Schmidtko et al. 2003, 2009; Luo et al. 2012; Lorenz et al. 2014). So far identified targets that are phosphorylated by cGKIα in DRG neurons include cysteine-rich protein 4 (CRP4; initially named CRP2, Schmidtko et al. 2008b), vasodilator-stimulated phosphoprotein (VASP), myosin light chains (MLC), inositol 1,4,5-triphosphate receptor 1 (IP3R1) (Luo et al. 2012), and possibly large-conductance Ca2+-activated K+ channels (BKCa) (Zhang et al. 2010; Lu et al. 2014).
However, consistent with the cellular distribution of cGKIα and NO-GC described above, double-immunohistochemical stainings confirmed that cGKI and NO-GC are not colocalized in DRGs and only partially colocalized in the spinal cord (Schmidtko et al. 2008a). This implicates that upstream mechanisms different from NO and NO-GC may activate cGKIα during pain processing. Indeed, several studies demonstrated that the particulate guanylyl cyclases GC-A and GC-B are colocalized with cGKIα in DRG neurons and mediate cGKIα activation after stimulation with natriuretic peptides (Schmidt et al. 2007; Kishimoto et al. 2008; Schmidtko et al. 2008a; Zhang et al. 2010). In addition, an alternate mechanism of cGMP-independent cGKIα activation has been recently discovered in DRG neurons: Oxidants such as hydrogen peroxide (H2O2) can cause interprotein disulfide bond formation between two cGKIα cysteine residues, rendering the kinase catalytically active, independently of cGMP (Burgoyne et al. 2007). Interestingly, H2O2-induced cGKIα disulfide bond formation was increased in DRGs after peripheral nerve injury, and knock-in mice with impaired H2O2 activation but normal cGMP activation of cGKIα demonstrated reduced neuropathic pain behaviors (Lorenz et al. 2014). Hence, both cGMP derived from particulate guanylyl cyclases and H2O2 derived from so far unidentified sources activate cGKIα in DRGs during pain processing. In contrast, NO and NO-GC seem to use targets different from cGKIα to mediate their pain-relevant effects in DRGs.
In a recent study, CNG channels were identified as a novel target of NO signaling during pain processing: Using in situ hybridization experiments, the CNG channel subunit CNGA3 was detected in inhibitory neurons of the dorsal horn and in DRG satellite cells. After hindpaw inflammation, CNGA3 expression was upregulated in the dorsal horn and in DRGs, and mice lacking CNGA3 (CNGA3−/−) showed increased inflammatory pain behaviors. Moreover, the pain hypersensitivity evoked by i.t. delivery of cGMP analogs and NO donors was increased in CNGA3−/− mice (Heine et al. 2011), indicating that CNGA3-positive CNG channels are a downstream target of NO signaling that contributes in an inhibitory manner to persistent pain processing. Further studies are required to identify additional downstream targets that mediate the pro- and antinociceptive effects of NO-mediated cGMP production.
3.3 S-Nitrosylation
A cGMP-independent mechanism of NO signaling is S-nitrosylation, i.e., the covalent and reversible attachment of NO to a reactive cysteine thiol (Hess et al. 2005). Several recent in vitro and ex vivo studies indicate that S-nitrosylation is a signaling mechanism of NO during pain processing (for review, see Tegeder et al. 2011). For example, whole-cell recordings of rat spinal cord slices revealed that NO may S-nitrosylate voltage-activated Ca2+ channels, thereby reducing glutamate release from primary afferent terminals (Jin et al. 2011). Unlike this antinociceptive mechanism, S-nitrosylation of actin was reported to ameliorate inhibitory postsynaptic currents in the spinal dorsal horn (Lu et al. 2011). In DRG neurons, NO was found to activate ATP-sensitive potassium channels by S-nitrosylation of cysteine residues in the SURI subunit, and this effect was not blocked by inhibitors of NO-GC or cGKI (Kawano et al. 2009). Furthermore, NO directly activated TRPV1 and TRPA1 channels in isolated inside-out patch recordings (Miyamoto et al. 2009), and it seems likely that this effect is also mediated by S-nitrosylation (Yoshida et al. 2006). In a recent proteomic approach using two-dimensional S-nitrosothiol difference gel electrophoresis and S-nitrosylation-site identification in spinal cord extracts, more than 50 proteins with modified S-nitrosylation in response to peripheral nerve injury were detected. The modified proteins are involved in synaptic signaling, protein folding and transport, mitochondrial function, and redox control (Scheving et al. 2012). The functional contribution of most of these proteins to pain processing is currently unknown; however, it seems very likely that S-nitrosylation essentially contributes to cGMP-independent NO signaling in the nociceptive system.
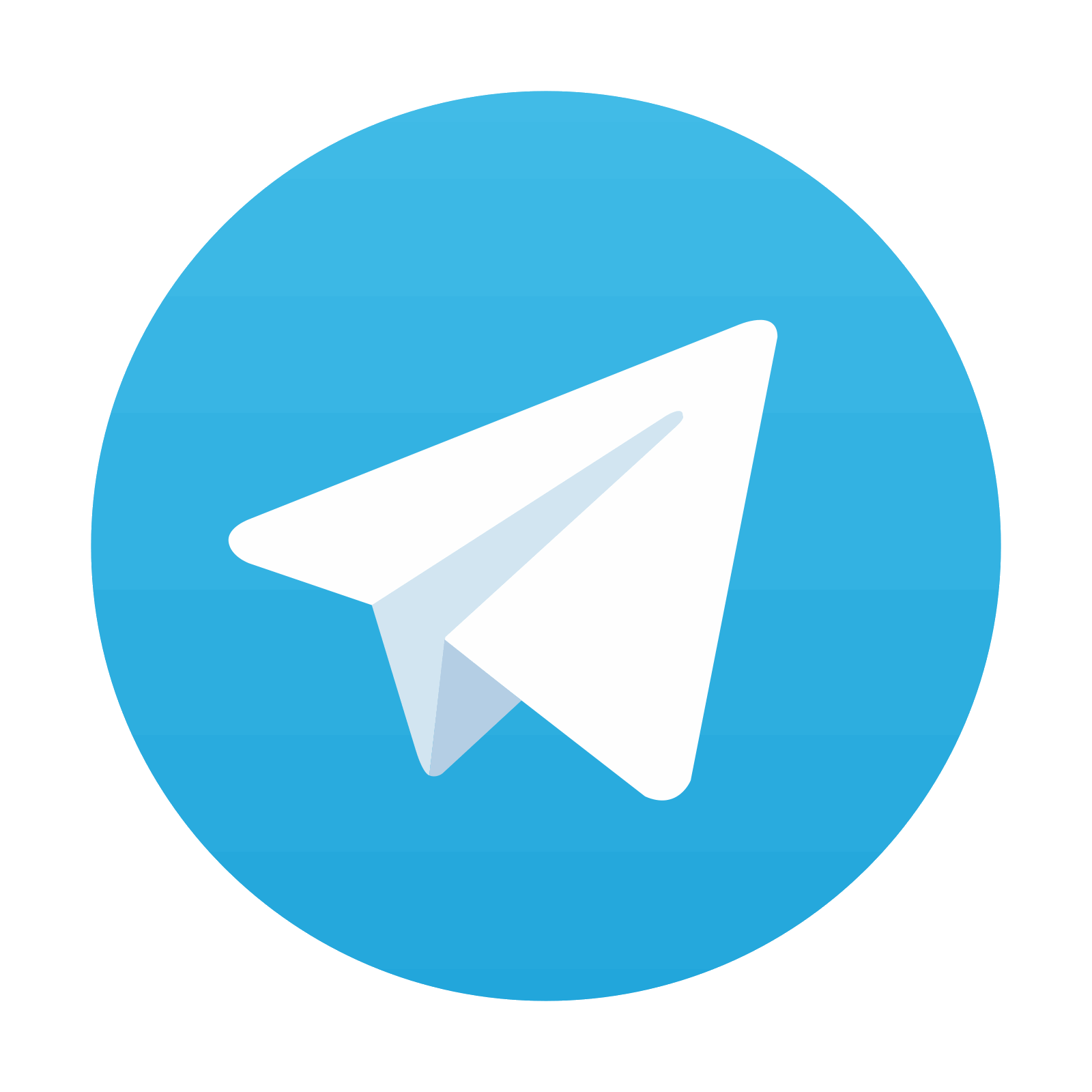
Stay updated, free articles. Join our Telegram channel
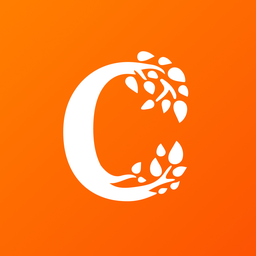
Full access? Get Clinical Tree
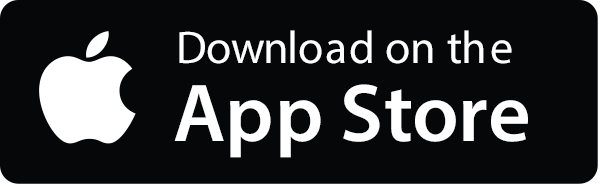
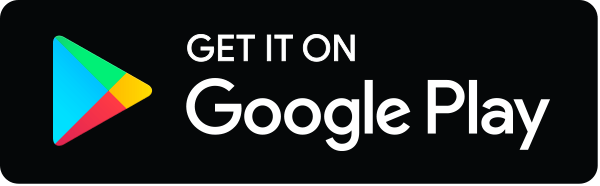