Mechanical Ventilation in the Neonatal and Pediatric Setting: Introduction
Respiratory disease in its various forms remains the most common cause of pediatric and neonatal morbidity and mortality. One of the most common reasons for admission to pediatric or neonatal intensive care units is the need for ventilatory support for acute or impending respiratory failure. The major challenge for these units is to deal with a very heterogeneous population of patients who are characterized by enormous differences in age and size and marked developmental changes in organ physiology during growth. In particular, the pediatric intensive care unit population is characterized by a wide variety of rare and unique medical problems that make large clinical trials, even on general topics such as ventilator support, very difficult to conduct.1,2 Despite worldwide daily use of mechanical ventilation in pediatric and neonatal intensive care units, many clinical and practical questions remain unresolved. Answers are often extrapolated from the results of adult studies. This may seem sensible for older children but is dangerous when applied to neonates, infants, and children up to the age of 12 years, because of developmental alterations in the physiology of their organ systems, particularly (but not only) their respiratory system.
Peculiarities in Physiology and Respiratory Mechanics
The considerable differences in respiratory physiology and anatomy between infants and adults3 explain why infants and young children have a higher susceptibility to more severe manifestations of respiratory diseases, and why respiratory failure is a common problem in neonatal and pediatric intensive care units (Table 23-1). The appreciation of the peculiarities of pediatric respiratory physiology is essential for correct management of critically ill and/or ventilated infants and children.
Cause | Physiologic or Anatomic Basis |
---|---|
Metabolism ↑ | ↑ O2 consumption |
Risk for apnea ↑ | Immaturity of control of breathing |
Resistance to breathing ↑ | |
Upper airway resistance ↑ | Nose breathing |
Large tongue | |
Airway size ↓ | |
Collapsibility ↑ | |
Pharyngeal muscle tone ↓ | |
Compliance of upper airway structures ↑ | |
Lower airway resistance ↑ | Airway size ↓ |
Collapsibility ↑ | |
Airway wall compliance ↑ | |
Elastic recoil ↓ | |
Lung volume ↓ | Numbers of alveoli ↓ |
Lack of collateral ventilation | |
Efficiency of respiratory muscles ↓ | Efficiency of diaphragm ↓ |
Rib cage compliance ↑ | |
Horizontal insertion at the rib cage | |
Efficiency of intercostal muscles ↓ | |
Horizontal ribs | |
Endurance of respiratory muscles ↓ | Respiratory rate ↑ |
Fatigue-resistant type I muscle fibers ↓ |
The basal metabolic rate is approximately twofold higher in infants than in adults (7 mL/kg/min at birth vs. 3 to 4 mL/kg/min in the adult). Hence, the normal resting state in infants is already one of high respiratory and cardiovascular activity. This means that infants have less metabolic reserve if O2 consumption needs to be increased during critical illnesses.
A considerable amount of maturation of the control of breathing occurs in the last few weeks of gestation and in the first few days of life, which explains the high prevalence of apnea in infants born prematurely.4 The breathing pattern of newborn, especially premature, infants is irregular with substantial breath-to-breath variability and periodic breathing at times, which increases the risk of prolonged, potentially life-threatening apnea under certain circumstances. The responses to hypercapnia or hypoxia are decreased and of variable sensitivity, making the young infant much more vulnerable to any noxious stimuli and disturbances of the respiratory control mechanisms.5
Ineffective breathing can lead to hypoxemia and bradycardia that may be severe enough to require the use of continuous positive airway pressure (CPAP) or intubation and mechanical ventilation, especially in the very preterm baby. Methylxanthines (such as caffeine) are effective in reducing the need of ventilator support for apnea of prematurity.4 Feedback from vagal stretch reflexes, rib cage muscles, and the changing mechanical state of the respiratory system during each breath influence respiratory activity. If inflation is small, there is little inhibitory vagal feedback. If inflation is excessive, inspiration is inhibited. This inspiratory–inhibitory reflex, the Hering-Breuer inflation reflex, is very potent in the preterm and less so in the term newborn and young infant during the first weeks of life.6 This inflation reflex facilitates passive measurement of respiratory mechanics, but may interfere with ventilator triggering. Babies may become apneic after a mechanical inflation, especially if an excessively prolonged inspiratory time (TI), is used.7 Fortunately, CPAP seems to enhance a baby’s ability to adjust to increased respiratory loads, possibly by the elimination of the Hering-Breuer deflation reflex.8
In the newborn, nasal breathing is obligatory, or at least strongly preferential, secondary to the configuration of the upper airways. The epiglottis is relatively large, floppy, and positioned high in the pharynx so that it is in contact with the soft palate, thereby favoring nasal over mouth breathing.9 The larynx, trachea, and bronchi are considerably more compliant than in the older child, thus making the infant’s airway highly susceptible to distending and compressive forces.10 Thus, with any obstruction of the upper airway, significant dynamic inspiratory collapse of upper airway structures can occur during forceful inspirations, which further adds to the obstruction already present. With lower-airway obstruction, forced expiratory efforts result in increased intrathoracic pressure and dynamic expiratory lower airway collapse further limiting expiratory flow. Positive end-expiratory pressure (PEEP) may help to stent collapsing airways during expiration in some patients (e.g., those with tracheobronchomalacia; Fig. 23-1).11
Figure 23-1
Bronchography in patient with tracheobronchomalacia without application of positive airway pressure (left) and with 10 cm H2O of positive airway pressure (right). Application of positive airway pressure produces stenting of the trachea and the bronchial tree. (Courtesy Quen Mok, Great Ormond Street Hospital for Children, London, UK.)
Small peripheral airways contribute approximately 50% to the total airway resistance in the infant lung (compared to approximately 20% in the adult). As airway diameter and length increase with age and growth, airway resistance falls tremendously from birth to adulthood.12 Therefore, diseases that affect the small airways and cause large changes in peripheral resistance may be clinically silent in an adult, but can cause significant problems in infants (e.g., bronchiolitis).
The airways of a child are relatively large in comparison with those of an adult, although in absolute terms they are small. Airway resistance in spontaneously breathing infants is normally 20–30 cm H2O/liter per second; values in intubated infants are 50 to 150 cm H2O/L/s, consequent to the diameter of the endotracheal tube (ETT).13 For many years it was thought that small infants could not breathe spontaneously through an appropriately sized ETT (because of the high resistance imposed by the small lumen), but this view is no longer maintained. Under normal tidal flow conditions, resistance imposed by a small tube is not substantially higher than that with larger tubes.14
Because of its shape, high compliance, and deformability, the contribution of the rib cage to tidal breathing is limited in newborns and infants. The ribs are horizontally aligned and allow for less anteroposterior movement of the chest wall and less efficiency of the intercostal muscles during respiration. The highly compliant chest wall is easily distorted, so that under conditions of respiratory impairment, much energy is wasted by sucking in ribs rather than fresh air. This paradox inward movement of the chest wall during inspiration is a common sign of almost any disorder causing respiratory distress in infants, but is most pronounced in upper-airway obstruction.
The elastic tissue in the septa of the alveoli surrounding the conducting airways provides the elastic recoil that enables the airways to remain open. Early in life there are few relatively large alveoli that provide little support for the airways, which are thus able to collapse easily. Addition of alveoli continues throughout early childhood by septal division, providing more elastic recoil and a decreased tendency for airway collapse with increasing age.15 Elastic recoil increases until adolescence and declines again with aging. Collateral pathways of ventilation (intraalveolar pores of Kohn and bronchoalveolar canals of Lambert) do not appear until 3 to 4 years of age,16,17 which excludes alveoli beyond obstructed airways to be ventilated by these alternate routes and predisposes the infant to the development of atelectasis.
The balance between the chest and lung recoil pressure that determines the static resting volume of the lung is set at lower lung volumes in infants compared with older children (Fig. 23-2).18 The highly compliant chest wall results in relatively low transpulmonary pressures at end-expiration that leads to an increased tendency for collapse of the small peripheral airways and ventilation inhomogeneity. The infant adopts several strategies to constantly establish lung volumes, including (a) a higher respiratory rate with insufficient time to exhale to the elastic equilibrium volume, (b) laryngeal adduction during exhalation to increase the resistance to airflow, and (c) constant postinspiratory diaphragmatic muscle activity (i.e., tonic diaphragmatic activity). A PEEP of 5 to 6 cm H2O is necessary to maintain end-expiratory lung volume in infants under neuromuscular blockade.19 The highly compliant chest wall also explains the generally high cardiovascular tolerance of infants to high airway pressures that are less efficiently transmitted to the pleural space. The low elastic recoil of the chest, together with a low percentage of slow-muscle fibers in both the diaphragm and intercostal muscles, explain why very little airway pressure is needed to expand the chest wall during inspiration in healthy babies.
Figure 23-2
Characteristics of lung and chest wall mechanics in newborns compared to adults. The compliant chest wall in the newborn leads to lower functional residual capacity (FRC) in the newborn. (Based on data published in Agostoni E. Volume-pressure relationships of the thorax and lung in the newborn. J Appl Physiol. 1959;14:909–913.)
Before birth, only approximately 10% of blood flow passes through the lungs as a result of high pulmonary vascular resistance, patent ductus arteriosus, open foramen ovale, and the low-resistance placental component to the systemic circulation. Pulmonary vascular resistance falls rapidly in the first minutes of life, but is still elevated and falls only gradually to normal levels over days to weeks. Under certain circumstances (e.g., birth asphyxia, chronic hypoxia, or neonatal septicemia with or without metabolic acidosis), pulmonary vascular resistance increases or remains high, leading to right-to-left shunting through the ductus arteriosus and sometimes even through the foramen ovale. This persistent pulmonary hypertension of the newborn (PPHN) leads to poor systemic oxygenation, as revealed by low postductal transcutaneous saturation levels or desaturation of all four extremities when the right-to-left shunt at the level of the foramen ovale is important.
High inflation pressures to recruit lung volume and improve oxygenation should be used with caution because of the risk of increased pulmonary vascular resistance by overdistension and compromised cardiac output. First-line treatment is directing toward known determinants of pulmonary vascular resistance (Table 23-2) such as acidosis or high interstitial pressures. Second-line therapy includes specific pulmonary vasodilators, such as inhaled nitric oxide (iNO)20–22 after excluding congenital heart disease with duct-dependent systemic circulation, which may deteriorate when pulmonary vascular resistance is lowered (e.g., total anomalous pulmonary venous return or hypoplastic left-heart syndrome).23
Increase in Pulmonary Vascular Resistance | Decrease in Pulmonary Vascular Resistance |
---|---|
High interstitial pressure | Low interstitial pressure |
High lung volumes (alveolar overdistension) | Normal lung volumes |
Low lung volumes (atelectasis) | |
Low alveolar PO2 | High alveolar PO2 |
Low arterial pH | High arterial pH |
Fetal hemoglobin is the predominant type of hemoglobin at birth and decreases steadily over the first 6 months. 2,3-Diphosphoglycerate (2,3-DPG) has a lower affinity to fetal hemoglobin and shifts the dissociation curve to the left. This facilitates loading and unloading of O2, ensuring, together with the high O2-carrying capacity of fetal hemoglobin, adequate tissue oxygenation despite low partial pressure of arterial oxygen (PaO2) values in utero (15 to 30 mm Hg). The predominance of fetal hemoglobin makes it possible, if necessary, to tolerate lower PaO2 values (but not really arterial oxygen saturation [SaO2]) better in early postnatal life than is possible later in life.
Preterm babies can maintain their growth in utero with a mean PaO2 of 3.2 kilopascals (kPa; approximately 24 mm Hg), equivalent to SaO2 of approximately 70%. In neonatal intensive care units, clinical practice has long been to keep oxygen levels in preterm newborns in line with those of term infants until neonatal morbidities induced by oxygen have been better understood. To date, there is insufficient evidence to suggest what would be the optimal SaO2 or PaO2 values in preterm infants to avoid potential oxygen toxicity while ensuring adequate oxygen delivery to tissues. There is, however, ample evidence to support the notion that a “restrictive” oxygen approach does more good than harm in preterm babies.24
Some Special Considerations for Ventilator Management in Neonates, Infants, and Children
The basic objectives of ventilator support remain the same from early life to adulthood25,26 but ventilator strategies, modes, and targets are not always fully identical to those established in adults.
Barotrauma zones, safe peak inspiratory pressure and PEEP, are less-well defined in the younger age group, especially the very premature infant. Neonatologists tend to limit peak inspiratory pressure to 30 cm H2O or lower; some favor the use of high-frequency oscillation ventilation (HFOV) in this age group. Studies investigating lung-protective ventilation in neonates have mainly focused on comparing high-frequency ventilation with conventional mechanical ventilation (CMV), leaving unanswered the important question as to whether reducing tidal volume or increasing PEEP is also lung protective.27 HFOV is best used with a high-lung-volume strategy, but offers no benefit over conventional ventilation when a lung-protective strategy is applied.28
A lung-protective strategy is commonly advocated similar to recommendations for adults following the publication of the ARDS Network trial.29 Recent observational cross-sectional studies, however, revealed that commonly used tidal volume (VT) varies between 6 and 10 mL/kg (mean: 8.3 ± 3.3 mL/kg) in children,2,30 and between 4 and 6 mL/kg (mean: 5.7 ± 2.3 mL/kg) in neonates.31 Interestingly, VT up to 10 mL/kg in children does not seem to be associated with increased mortality. Moreover, higher VT within this range is associated with more ventilator-free days, particularly in patients with less-severe disease.30 In a recent prospective, multicenter, observational study, higher maximum and median tidal volumes were associated with reduced mortality, even when corrected for severity of lung disease.32 There is even less data available for neonates than for children to confirm or reject the hypothesis that small-volume ventilation, VT of 4 to 6 mL/kg, is the best lung-protective approach.27
Overventilation and hypocapnia have been shown to contribute to adverse neurodevelopment in preterm infants and also in the neonate with hypoxic ischemic encephalopathy.33–35 Any rapid reduction in partial pressure of arterial carbon dioxide (PaCO2), which may occur with surfactant therapy or change in ventilator mode, may lead to cerebral vasoconstriction, increasing the risk of cerebral hemorrhage and/or leukomalacia. Both extremes and fluctuations of PaCO2 are associated with severe intraventricular hemorrhage. It may be prudent to avoid extreme hypocapnia and hypercapnia during the period of risk for intraventricular hemorrhage in preterm infants. Both minimum partial pressure of carbon dioxide (PCO2) and cumulative PCO2 less than 35 mm Hg are associated with poor outcome in neonates with hypoxic ischemic encephalopathy.34
Modern neonatal ventilator modes can target a set tidal volume as an alternative to traditional pressure-limited ventilation. Volume-targeted ventilation (VTV) aims to produce a more stable VT so as to reduce lung damage and stabilize PaCO2. Infants receiving VTV had lower rates of death and chronic lung disease compared with infants ventilated using pressure-limited ventilation modes.36,37 Further studies are needed to identify whether VTV modes improve neurodevelopmental outcomes and to compare and refine VTV strategies.
VTV has some technical limitations. First, the displayed VT can be misleading in the presence of a high ETT leak. Such leak is common in neonatal practice where uncuffed tubes are commonly used and may be noted in approximately 75% of all ventilated neonates, usually without greater clinical relevance.38 Leaks are associated with an inability to detect the patient’s inspiratory effort or autotriggering of the ventilator. The latter also occurs if tubing is not kept dry and if there is water accumulation in the expiratory limb. This complication is more common in the neonate and young infant. Second, inaccurate VT delivery under specific conditions, especially when rapid changes in respiratory system mechanics occur, has been described with various neonatal ventilators that offer VTV. Such discrepancies between the set VT and the delivered inflations can be harmful in clinical situations, especially in newborns. Their clinical relevance needs to be clarified with safety studies in the neonatal population, before the “uncritical” use of such new hybrid modes could be advocated for all neonates and for various clinical conditions.39
There is a tendency towards using very short inspiratory times in premature infants with respiratory distress syndrome (RDS) secondary to the very low compliance of the lungs. An increase in inspiratory time may sometimes result in lower peak inspiratory pressures and more homogenous ventilation. A good knowledge of respiratory mechanics is helpful to decide on the best settings. In addition, there exists the erroneous belief that expiratory times have to be longer than inspiratory times. While this is the case in obstructive airways disease, it does not apply to the healthy or the stiff lung in neonates and children.
The small and narrow ETTs are prone to mucus plugging and kinking. Many clinicians believe that for an infant or young child, breathing through a small ETT is equivalent to breathing through a straw, thereby imposing an unacceptable work of breathing. This notion is contrary to both clinical observation and physiology.14,40 A 3-kg infant accepts a 3-mm inner-diameter ETT, whereas a 60-kg adult can tolerate a 9-mm inner-diameter ETT—a 20-fold increase in body size but only a threefold increase in ETT size. The narrower tube is irrelevant because it is shorter and because lower flows are generated by the infant compared to the adult. The net effect is that the infant is breathing through a hose rather than a straw when compared to the adult. Hence, if an infant or young child cannot sustain a spontaneous breathing trial on CPAP or a T-piece for several hours, he or she is as likely to fail extubation as when pressure support is applied. Furthermore, the addition of pressure support is likely to mask respiratory insufficiency and contribute to a higher failed extubation rate.41
Tracheotomy is withheld for a longer period in critically ill children than in adults. Infants tolerate prolonged intubation much better than adults, although complications, such as subglottic stenosis,42 can occur. Furthermore, indications for tracheotomy are different between children and adults: Tracheotomy is more often appropriate for bypassing congenital or acquired upper-airway obstruction than for supporting long-term mechanical ventilation.43
There is probably no medical device other than closed-suction systems that has been as enthusiastically accepted in intensive care unit practice without the requirement to prove benefit in a randomized controlled trial. There is still insufficient evidence to decide between endotracheal suctioning with or without disconnection.44 In the injured lung, however, closed suction is much less effective than open suction, irrespective of the type of secretions.45 The negative effects of suction on lung volume, heart rate, and SaO2 are transient and highly variable with both open-suction and closed-suction methods in spontaneously breathing infants.46 This suggests that it is time to reassess the role of open suction, especially in ventilated infants with narrow tubes and respiratory disease resulting in large amounts of copious secretions.
Common Clinical Conditions
RDS is characterized by pulmonary surfactant deficiency and transudation of plasma proteins into the alveolar spaces. It typically occurs in preterm infants, and its severity correlates with the degree of immaturity of the lungs and is aggravated by concomitant infection, oligohydramnios, and a variety of other factors. The results are stiff lungs and a tendency to atelectasis. Initial ventilator management aims to recruit collapsed alveoli, restore functional residual capacity, and achieve adequate alveolar ventilation. Technical and pharmacologic advances, with improved understanding of pathophysiology and causes of lung injury, have significantly enhanced the ventilator management of preterm infants with RDS. Ventilator modalities have shifted towards early noninvasive ventilation and decreased and shortened use of invasive forms of ventilation in most preterm infants.
Nasal CPAP applied by various devices has become the primary respiratory support for RDS. When used early, it reduces respiratory failure, the need for surfactant, and the duration and invasiveness of respiratory support without impairing neonatal outcome.47 It therefore offers a valuable alternative to intubation and surfactant in preterm infants.48 It has not, however, proven to allow for better outcome than intubation and mechanical ventilation in preterm infants.49 Nasal CPAP improves respiration in preterm infants by increasing functional residual capacity and chest wall stability, as well as decreasing upper-airway collapsibility and upper-airway resistance.50
Recently, heater-humidifier devices that use novel methods for conditioning respiratory gases from an external source have been introduced. The addition of sufficient warmth and high levels of humidification to a gas has enabled the use of higher flow rates from a nasal cannula. High-flow nasal cannula can be used to provide high concentrations of O2 and may deliver PEEP. At present, there is insufficient evidence to establish the safety or effectiveness of high-flow nasal cannula as a form of respiratory support in preterm infants. When used following extubation, high-flow nasal cannula seems to result in a higher rate of reintubation than does nasal CPAP.51
When positive-pressure ventilation was first introduced in newborn infants with RDS, the use of high peak inspiratory pressures was associated with high mortality rates, air leaks, and the development of bronchopulmonary dysplasia (BPD).52 In the 1970s, the incidence of pneumothoraces was reduced by adopting a strategy using long TI values and slower rates.53 This strategy was widely adopted and only given up after the benefit of PEEP was better understood.54 Administration of exogenous surfactant and advancements in ventilator technology, which decrease patient–ventilator asynchrony, have further improved the management and outcome of RDS. It is now widely accepted that severe RDS, characterized by poorly compliant lungs and very short time constants, is best managed with short TI values (0.26 to 0.34 seconds), rapid rates (60/breaths/min or more), and PEEP.55,56 Such general recommendations help in avoiding major errors when initiating mechanical ventilation in infants suffering from severe RDS. Nevertheless, inspiratory and expiratory time, respiratory frequency, and PEEP need to be customized to an individualized assessment of a patient’s respiratory mechanics, which change over the course of disease or with therapeutic interventions such as surfactant administration. The availability of low-dead-space-flow sensors enables continuous measurement of VT, and eliminated much of the guesswork in setting the ventilator. Volume-targeted modes have now become increasingly popular in the neonatal intensive care unit to improve the stability of ventilation and to reduce unnecessarily high peak pressures.37 This strategy aims at reducing PaCO2 fluctuations in very preterm infants (although it is not always as successful as hoped57,58), which are associated with the development of intraventricular hemorrhage and leukomalacia. Hence, much emphasis is placed on using ventilator strategies that should best guarantee a constant PaCO2 and at the same time use of the lowest fractional inspired oxygen concentration (FIO2) to achieve sufficient tissue oxygenation.
The underlying pathophysiology, decreased lung compliance, high chest wall compliance, and dynamically maintained functional residual capacity above closing volume make RDS a perfect candidate for an open-lung strategy and use of HFOV. Despite many studies of HFOV versus conventional ventilation (and three meta-analyses of these trials) involving 3652 infants, neonatologists remain unsure about the potential benefits and harms of HFOV for support of preterm infants with surfactant-deficient lungs. The Prevention of Ventilator Induced Lung Injury Collaborative Group (PreVILIG collaboration) recently concluded that HFOV seems equally effective to conventional ventilation in preterm infants.59 Current results do not support selection of preterm infants for HFOV on the basis of gestational age, birthweight for gestation, initial lung disease severity, or exposure to antenatal corticosteroids.59 Why data from animal models predicted a larger effect size than that observed in human infants remains an unanswered question. Recent data, however, support the usefulness of first-intention HFOV strategy in managing selected subpopulations, such as very-low-birthweight newborns complicated by severe RDS not antenatally treated with glucocorticoids.60
Exogenous surfactant therapy has been part of routine care of preterm neonates with RDS since the early 1990s. Animal-derived exogenous surfactants are the present treatment of choice, with few adverse effects. The few adverse effects are largely related to changes in oxygenation and heart rate during surfactant administration.61 The optimal dose is usually 100 mg/kg. Both prophylaxis and early treatment are successful in infants with established RDS, but prophylaxis appears to produce greater benefit.62 The concept of using surfactant early in patients with low oxygen needs (FIO2 <0.45), combined with the so-called INSURE approach (intubation-surfactant-extubation to nasal CPAP), has decreases the need for mechanical ventilation, incidence of BPD, and air leak syndromes as compared to subsequent use of INSURE when FIO2 exceeds 0.45.47,63
Persistent fetal circulation is a complication of RDS in preterm infants. At present, there is no clearcut evidence in favor of using iNO for preterm infants requiring mechanical ventilation.64 Clinicians continue to make case-by-case decisions for the treatment of preterm infants with hypoxia unresponsive to other therapies.65
PPHN is the result of elevated pulmonary vascular resistance to the point that venous blood is shunted to some degree through fetal channels (e.g., ductus arteriosus and foramen ovale) into the systemic circulation. By bypassing the lungs, it causes systemic arterial hypoxemia. It is associated with (a) pulmonary parenchymal disease such as RDS or meconium aspiration and (b) hypoplasia of the lungs, most often in the form of diaphragmatic hernia. It (c) also can occur without an evident cause.
Traditionally, these infants have been hyperventilated to achieve mild hypocapnic alkalosis in an attempt to attenuate hypoxic pulmonary vasoconstriction. iNO is an approved adjunct to improve oxygenation in term and preterm infants with severe hypoxemic respiratory failure secondary to PPHN and has been shown to reduce the need for extracorporeal membrane oxygenation.66 Alveolar recruitment should be optimized to achieve best iNO effects on oxygenation.23 Hence, HFOV is an ideal method to combine with iNO.
Meconium aspiration syndrome affects mature infants and is characterized by a mixture of inflammatory pulmonary disease, secondary surfactant deficiency, obstruction of small airways, and pulmonary hypertension. The result is a very inhomogeneous lung with areas of atelectasis and hyperinflation. In terms of pathophysiology and ventilator management, meconium aspiration syndrome resembles acute respiratory distress syndrome (ARDS) of pulmonary origin, favoring the use of similar ventilator strategies (open lung concept, permissive hypercapnia, and so on). Despite the introduction of innovative ventilatory treatments for this disease over the last two decades (e.g., surfactant-lavage, HFOV, iNO, extracorporeal membrane oxygenation), most infants can be successfully managed with CPAP or mechanical ventilation alone.67,68
Congenital diaphragmatic hernia (CDH) is characterized by lung hypoplasia, surfactant deficiency, and an extremely reactive hypoplastic pulmonary vascular system. The hypoplastic lungs are very vulnerable to injury from aggressive ventilation with high inspiratory pressures. Treatment is no longer focused on immediate surgery, but rather on delaying repair until all reversible problems are resolved (i.e., stabilization period).69,70 The concept of delaying repair until pulmonary hypertension resolves and the patient is hemodynamically stable has increased survival from below 50% to as high as 80%.69,71 Although high airway pressures may be required with secondary parenchymal injury to ensure adequate oxygenation, special care is necessary to avoid alveolar distension in the setting of pulmonary hypoplasia. Neither the use of HFOV nor early rescue extracorporeal membrane oxygenation, however, has significantly improved outcome in CDH patients, the best survival rates with these options are approximately 80%.72–77 The discussion on optimal treatment strategies for CDH, in addition to the commonly accepted need for early lung-protective ventilation to avoid lung injury, is not closed.78
Two distinct patterns of disease occur in infants with virus-induced respiratory failure.79,80 The primary causative agent is respiratory syncytial virus. The most frequent pattern is acute bronchiolitis characterized by an obstruction of small airways with air trapping and a moderate degree of parenchymal disease from atelectasis. This leads to increased respiratory resistance, a prolonged time constant, intrinsic PEEP, and decreased respiratory compliance.81 Radiographic findings include hyperinflation, perihilar infiltrates, and atelectasis. The second pattern, which affects approximately 25% to 30% of infants with respiratory syncytial virus-induced respiratory failure, consists of severe restrictive parenchymal disease (usually termed respiratory syncytial virus-pneumonia). Typically, respiratory compliance and lung volumes are decreased markedly without significant airway obstruction or air trapping. Alveolar consolidation is the main radiographic feature. This subgroup also usually fulfills the criteria of ARDS. Patients require prolonged ventilation compared with a brief duration (4 to 8 days) for the bronchiolitic pattern.79,80
No consensus exists on optimal ventilator strategy. Nasal CPAP appears useful in the early stages of severe bronchiolitis by decreasing the work of breathing needed to overcome the intrinsic PEEP. The evidence supporting the use of CPAP to reduce PCO2 and respiratory distress in bronchiolitis, however, is of low methodologic quality, reporting only short-term effects,82 and there is no conclusive evidence that CPAP reduces the need for intubation.83,84 More recently, high-flow nasal cannula, if used early in the treatment of severe bronchiolitis, appears to reduce the need for intubation in infants with viral bronchiolitis.85
Pressure-controlled ventilation (PCV) is the mode used most commonly in virus-induced respiratory failure because the decelerating flow pattern achieves a lower mean airway pressure than volume-controlled ventilation (VCV). Most patients need peak inspiratory pressures of 25 to 35 cm H2O to achieve adequate ventilation. Infants with obstructive disease have long expiratory time constants. They are best ventilated with slow rates and inspiratory-to-expiratory (I:E) ratios of at least 1:3 to prevent breath “stacking” and further hyperinflation. Conversely, patients with restrictive disease may require faster rates and lower I:E ratios. Permissive hypercapnia, to avoid high peak inspiratory pressures and barotrauma, can be used with both pathophysiologic patterns.
Obstructive patients may do poorly with HFOV, although this statement is not as absolute as often thought.86 Patients with predominant restrictive disease, however, are better candidates for HFOV.
The causes of ARDS in pediatric patients are the same as in adults, although viral or bacterial respiratory infections are more common in children.79 Ventilator strategies are the same as in adult patients and include high PEEP and limiting peak inspiratory pressure to below 30(−35) cm H2O. Lung-protective ventilation strategies are commonly recommended, although there is doubt as to the best VT.
Although no single ventilation mode has proven superior, the most common modes are PCV and HFOV,2,30,87 despite the absence of solid data on the use of HFOV in pediatric patients beyond the neonatal period. Prone positioning is used commonly, but without proven benefit.88,89 iNO cannot be recommended for routine use, although it is used as rescue therapy (for a short time) in the very early stages of life-threatening hypoxemia and severe pulmonary hypertension.
Most complications in patients with asthma receiving ventilation occur during or immediately after intubation. They result largely from gas trapping that causes hypotension, O2 desaturation, pneumothorax, and cardiac arrest. Institution of positive-pressure ventilation in patients with asthma alters cardiocirculatory and respiratory dynamics dramatically, leading to diminished venous return and hypotension.
The optimal ventilator mode for status asthmaticus is not established. Randomized, controlled trials comparing different modes in pediatric asthma patients are virtually impossible.90 Most clinicians prefer pressure-limited ventilation, keeping peak inspiratory pressure below 35 to 40 cm H2O and accepting hypercapnia.91 Because of their decelerating flow pattern, PCV and pressure-regulated VCV allow for lower peak inspiratory pressures but result in higher mean airway pressures than VCV with identical VT. Ventilator rates are set well below normal and require an extremely long expiratory and reasonably long inspiratory time. Externally applied PEEP should be, in general, just below auto-PEEP to decrease trigger work but not increase hyperinflation. Duration of mechanical ventilation for status asthmaticus is usually of 1 to 4 days. Children with rapid-onset near-fatal asthma may have a shorter duration of ventilation than children with asthma that progresses slowly to respiratory failure.92
HFOV, pressure-support ventilation (PSV), and noninvasive ventilation have been tried in status asthmaticus. HFOV is believed to be contraindicated in children with severe airflow obstruction, although recent experience challenges this belief.93 PSV enables active exhalation, which may decrease hyperinflation. PSV allows patients to determine their own respiratory pattern (rate, TI, and VT) and may decrease patient–ventilator dyssynchrony. PSV decreases work of breathing by partially unloading the respiratory muscles. Careful selection of PEEP decreases the work of triggering during PSV. PSV of 22 to 37 cm H2O has been used successfully in children with asthma requiring full or near-full support, resulting in rapid improvement in gas exchange.94 Noninvasive PSV is often poorly tolerated without sedation, and its role in avoiding intubation in children with status asthmaticus remains unclear.95,96
Correct Timing of Extubation
Avoidance of unnecessary weaning delays is important for reducing the risk of nosocomial infection, ETT damage to the airway, development of chronic lung disease in neonates, and prolonged dependency on narcotic drugs.97,98 Aggressiveness with early weaning, however, must be balanced against the risk of extubation failure that occurs in 2% to 20% of children41,99 and up to 40% of neonates,100 and which is associated with increased mortality.101
Several indices have been developed to predict successful weaning and extubation. Although these indices have been used in research studies, they have not found widespread use in clinical care, likely because of their complexity and lack of proven benefit over clinical judgment. A trial of spontaneous breathing with a T-piece or the use of 10 cm H2O of PSV for up to 2 hours has been successful in predicting extubation failure in children.102 A modified rapid shallow breathing index, also known as frequency-to-tidal-volume ratio, and the compliance, rate, oxygenation, and pressure index has been proposed for weaning prediction in children.103
There is fertile ground for future research to identify simple predictors of extubation readiness that would likely shorten the duration of ventilation, decrease length of intensive care unit stay, and potentially reduce ventilator-induced lung injury.41 Table 23-3 lists proposed clinical and laboratory criteria for weaning failure during 2 hours on CPAP of 5 cm H2O or a T-piece. The single most common cause of extubation failure in children, however, is upper-airway obstruction, which is difficult to predict with an ETT in place. The air-leak test (an audible air leak when airway pressure ≥25 cm H2O), although often recommended, has repeatedly been shown to have low sensitivity for testing extubation readiness.104–106
Proposed criteria for failure of extubation readiness during 2 hours on CPAP <5 cm H2O or T-piece |
---|
|
Extubation failure is more frequent in children with chronic respiratory disorders, neuromuscular and chronic neurologic disorders, and upper-airway problems.101 The most common cause of extubation failure in preterm infants is apnea with bradycardia.107
Developing reliable thresholds for indices for weaning extremely low-birthweight preterm infants has proven equally difficult as for older children.108 The percentage of time spent below a target value of spontaneous expiratory minute ventilation (<125 mL/min/kg) during a 2-hour CPAP trial appears promising but requires further testing.109,110 Another option might be a spontaneous breathing trial of 3 minutes on CPAP after minimal ventilator settings have been reached.111
The most common approach to weaning infants and children is gradual reduction of ventilator support by decreasing rate and airway pressures during synchronized intermittent mandatory ventilation (SIMV). Other weaning methods include PSV and volume-support ventilation. Both methods involve patient-triggered pressure support. With PSV, the level of PSV is adjusted gradually by the physician to a minimal level that achieves an acceptable breathing pattern. With volume-support ventilation, the level of PSV is adjusted continuously by the ventilator to achieve minimum minute ventilation. It is common practice to extubate infants and children from a low level of ventilator support. Gradual weaning is often unnecessary after the underlying disorder has resolved.102,112
Today, many modes have been developed to facilitate weaning by reducing work imposed by the respiratory apparatus.113,114
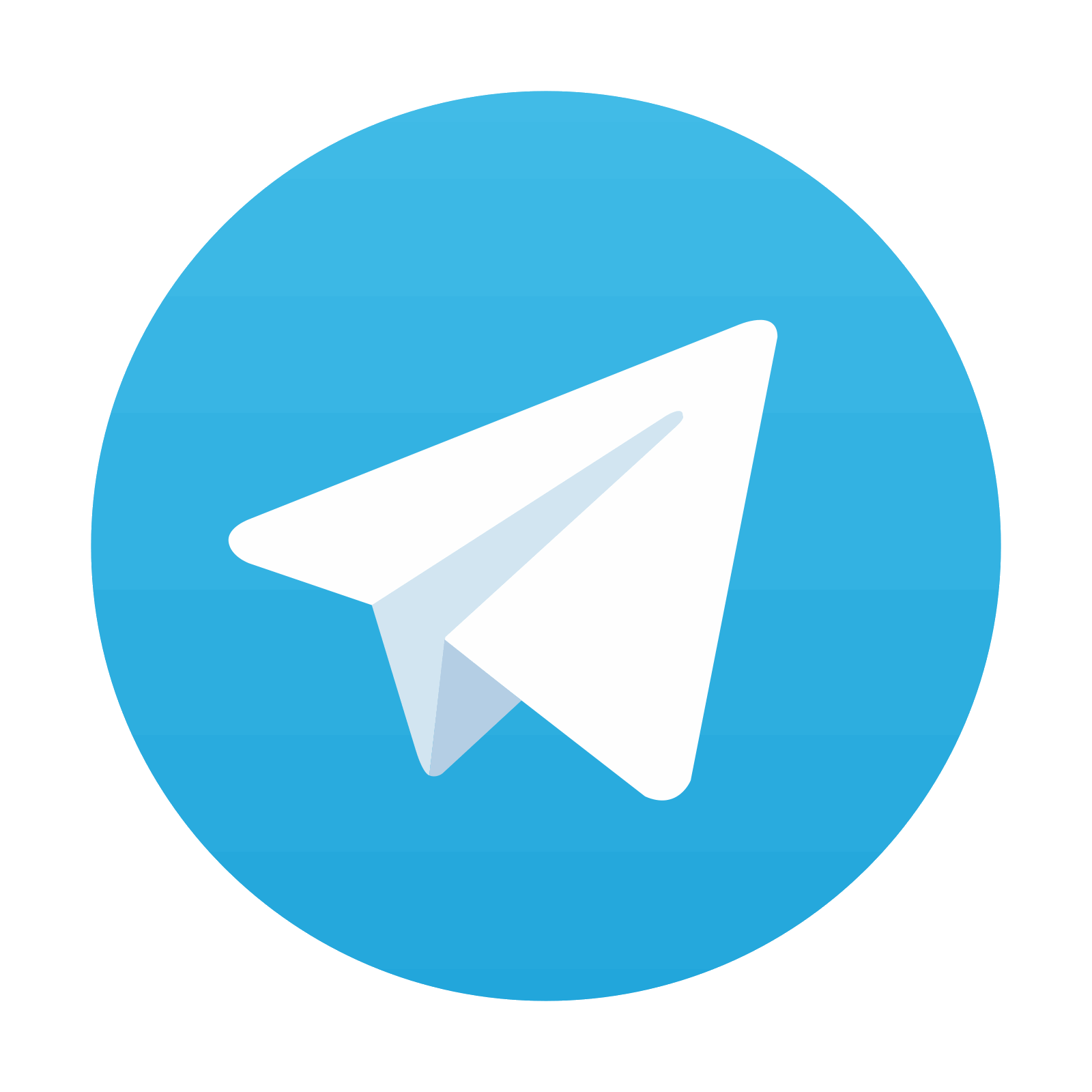
Stay updated, free articles. Join our Telegram channel
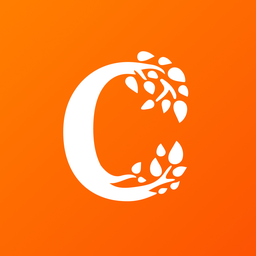
Full access? Get Clinical Tree
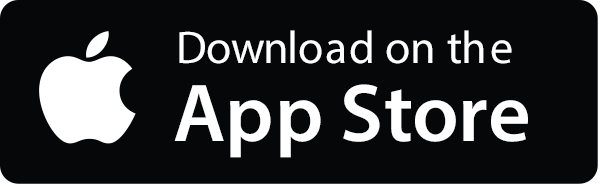
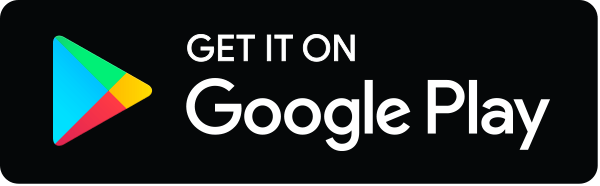
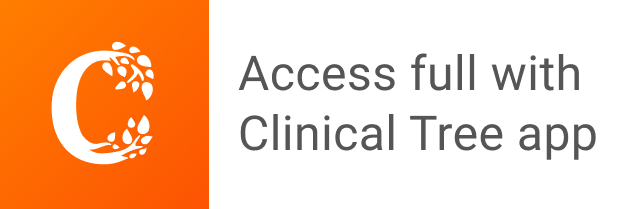