Assist-Control Ventilation: Introduction
Volume assist-control ventilation (ACV) is a ventilator mode in which the machine delivers the same tidal volume during every inspiration, whether initiated by the ventilator or by the patient. This occurs regardless of the mechanical load on the respiratory system and no matter how strenuous or feeble the inspiratory muscle effort. Current data indicate that ACV is still the most frequently used mode in intensive care units (ICUs).1 Nowadays, the main reason for patients being admitted to an ICU is the need for mechanical ventilation,2 and the most common reason to initiate mechanical ventilation is acute respiratory failure.1,3,4 Approximately 60% of intubated, ventilated patients receive ACV.5 This percentage is similar for patients ventilated for decompensated chronic obstructive pulmonary disease (COPD),5 and even higher for those ventilated for acute respiratory distress syndrome (ARDS).6
Basic Principles
In ACV, mechanical breaths can be triggered by the ventilator or the patient. With the former, triggering occurs when a certain time has elapsed after the previous inspiration if the patient fails to make a new inspiratory muscle effort (Fig. 6-1). The frequency at which time triggering takes place is determined by the backup rate set on the ventilator. When patients trigger a mechanical breath, their spontaneous inspiratory effort is sensed by the machine, usually as a change in airway pressure or airflow. When such a change crosses the trigger-sensitivity threshold, the ventilator delivers the preset tidal volume. Chapter 3 provides a detailed explanation regarding the working principles of ventilators.
Figure 6-1
(From top to bottom) Tracings of airflow (FLOW), airway pressure (Paw), esophageal pressure (Pes), gastric pressure (Pga), and tidal volume (VOLUME). Each mark on the time axis denotes 1 second. These recordings were obtained in a passively ventilated patient. Each breath is time-triggered.
Mechanical breaths have precise mechanisms for being initiated (trigger variable), sustained (limit variable), and stopped (cycle variable). These are known as phase variables.7 In ACV, the mechanical breaths are limited by volume and/or flow and cycled by volume or time. The inspiratory flow-shape delivery is usually a square (constant) during ACV, although some ventilators also permit sinusoidal and/or ramp (ascending or descending) gas flows.
Physiologic Effects
Mechanical ventilation is a lifesaving supportive treatment that improves gas exchange and decreases the mechanical workload of the respiratory muscles while buying time for the patient to recover. The way mechanical ventilation is used is central to its short-term and long-term effects. Ventilator settings are a major determinant of the physiologic and clinical effects of ACV. Chapters 36 and 37 address the physiologic effects of ACV on gas exchange and cardiovascular function.
In every assisted mode, the ventilator responds to a patient’s inspiratory effort. Both pressure-triggering and flow-triggering systems of modern ventilators offer high performance, and the differences are small in terms of the added work of breathing. A bench study comparing the performance of new-generation ventilators versus old-generation ventilators revealed that triggering function, pressurization capacity, and expiratory resistance are globally similar, thus suggesting that a technological ceiling has been reached.8
Marini et al9 reported that decreases in trigger sensitivity increased the work of breathing. Although decreasing the inspiratory flow rate from 100 to 80 L/min did not affect the effort to breathe at a moderate minute ventilation (12 L/min), it increased the work expenditure significantly when the minute ventilation was doubled.9 When inspiratory flow was reduced to 40 L/min and thus did not match the subject’s demand, the work of breathing increased by 50%. Marini et al10 later analyzed the inspiratory work at two inspiratory flow settings, 60 and 100 L/min, in twenty patients. Tidal volume was unchanged. The patients’ work per liter of ventilation at both ACV inspiratory flow settings represented approximately 60% of the work dissipated during spontaneous breathing. Dead space was added in half the patients and led to marked increases in muscle effort. Patients’ work of breathing did not correlate with minute ventilation, although it was highly correlated with respiratory drive and muscle strength. A decrease in inspiratory flow to 40 L/min (in five patients) led not only to an increase in effort but also to premature expiratory efforts, encroaching on the ventilator’s inspiratory time. In total, these data demonstrated that inspiratory muscle effort persists throughout the inflation and that a substantial amount of muscle work is dissipated during ACV.
Ward et al11 analyzed the inspiratory muscle effort at several inspiratory flow rates between 25 and 65 L/min and confirmed the findings by Marini et al. Cinella et al12 compared ACV and assist pressure-controlled ventilation (PCV) and used two tidal volume settings (12 and 8 mL/kg) with an inspiratory time of 1 second and no end-inspiratory pause. At high tidal volumes, no differences were observed between the two modes in terms of breathing pattern or indices of inspiratory muscle effort. When a tidal volume of 8 mL/kg was used (and thus inspiratory flow decreased with ACV), differences arose. Respiratory rate and occlusion pressure (a measure of respiratory motor output) tended to be higher with ACV, and indexes of inspiratory muscle effort showed a marked increase as compared with PCV. In the second part of this study,12 the authors compared the effects of ACV and assist PCV using a fixed tidal volume (8 mL/kg) and two different settings for inspiratory time: 1 second and 0.6 second with no pause. With the longer inspiratory time, the differences were the same as stated previously. When inspiratory time was reduced and thus inspiratory flow increased during ACV, the differences between the modes virtually vanished. The study showed that both modes unloaded the respiratory muscles equally, provided that the inspiratory flow rate was set appropriately during ACV. These data confirm the importance of maintaining an inspiratory flow rate high enough to satisfactorily unload the respiratory muscles and also point out that moderate to low tidal-volume ventilation using high flow rates results in a short inspiratory time, which may not be optimal for some patients. The duration of diaphragmatic contraction was unaffected by the ventilator settings and always was shorter than 1 second.12 Thus, it appears that the effects of high airflow settings on muscle unloading are mainly exerted at the very beginning of inspiratory efforts. Similar results were obtained by McIntyre et al13 when comparing ACV with a pressure-limited volume-guaranteed dual mode. These authors, however, suggested that the pressure-limited breaths could reduce patient–ventilator flow dyssynchrony. These physiologic effects were confirmed in a subsequent study performed by the same group.14
A number of investigators have shown that patients12,15–17 and healthy individuals18,19 react to an increase in inspiratory flow with an increase in respiratory rate when tidal volume is kept constant. In these circumstances, the imposed ventilator inspiratory time shortens as flow increases. This leads to a decrease in neural inspiratory time. When tidal volume is increased by lengthening the duration of inspiratory flow, neural expiratory time increases, and respiratory rate tends to decrease. These changes have opposite effects on respiratory rate. The mechanisms explaining these responses are complex and include the Hering-Breuer reflex (inhibits inspiration and prolongs expiration), reflexes mediated by vagal mechanoreceptors, and perhaps consciousness-mediated reflexes.20–22
Airflow-induced changes in breathing pattern carry important clinical implications, especially in patients with dynamic hyperinflation. Because inspiratory time is made up of the time of flow delivery and inspiratory pause, Laghi et al17 hypothesized that a decrease in ventilator inflation time would cause an increase in rate. In ten noninvasively ventilated stable patients with an obstructive airway disease, the investigators increased flow at constant tidal volume and decreased the inspiratory pause, keeping inspiratory flow and tidal volume constant. When inspiratory time was decreased (by increasing flow from 30 to 90 L/min), respiratory rate and expiratory time increased significantly. Intrinsic positive end-expiratory pressure (PEEP) diminished significantly despite the increase in respiratory rate. When inspiratory time was decreased by shortening the inspiratory pause, both respiratory frequency and expiratory time increased significantly. Again, intrinsic PEEP decreased significantly. Additionally, the higher inspiratory flow rates also decreased respiratory drive and inspiratory effort. These results suggest that imposed ventilator inspiratory time duration determines the respiratory rate and that the strategies that reduce ventilator inspiratory time, although accompanied by an increase in respiratory rate, also prolong the time for exhalation, thus decreasing intrinsic PEEP.
Mechanical ventilation per se can induce respiratory muscle damage,23–25 and patients appear to exhibit diaphragmatic weakness after a period of mechanical ventilation.26 The term ventilator-induced diaphragm dysfunction was coined to express the decrease in the force-generating capacity of the diaphragm that results after a period of passive controlled mechanical ventilation.27 Le Bourdellès et al28 showed that anesthetized, passively ventilated rats had lower diaphragmatic weight and a reduction in their force-generating capacity in comparison with spontaneously breathing control animals. Anzueto et al29 studied sedated, paralyzed baboons under ACV for 11 days. Endurance time decreased over this period, and transdiaphragmatic pressure diminished by 25%, suggesting that the duration of passive ACV is also a relevant factor.
Sassoon et al30 showed that 3 days of passive ventilation in rabbits led to a progressive decrease in the force-generating capacity of the diaphragm in comparison with control animals who received the same total amounts of sedatives but were breathing spontaneously. They also showed that significant diaphragmatic myofibril damage had occurred. Other authors have reported similar data.31,32 Several investigators33–36 have begun to elucidate the complex cellular, molecular, and gene expression mechanisms underlying passive ventilation-induced respiratory muscle damage. Such mechanisms include, among others, decreased protein synthesis, increased proteolysis, oxidative stress, and alterations in cytosol calcium metabolism.27
Subsequent findings by Sassoon et al37 carry important clinical implications. The authors found that ACV, as compared with passive ACV, can attenuate markedly the decrease in diaphragmatic force induced by total inactivity in rabbits. Another investigation with clinical ramifications has shown that passive ACV improves diaphragmatic force production in rats challenged with intravascular endotoxin as compared with equally challenged spontaneously breathing animals.38
An interesting issue is the combined effects of certain drugs (e.g., corticosteroids) on diaphragmatic function during passive ventilation. Maes et al39 studied the effects of corticosteroid administration in rats (a single injection of 80 mg methylprednisolone/kg) on diaphragm function. The animals were ventilated with passive ACV for 24 hours. The main finding of this investigation was that a very high dose of corticosteroids protected the diaphragm against the deleterious effects of passive ACV. The diaphragm of treated animals maintained force, fiber dimension, and myogenin protein levels, whereas the diaphragm of nontreated animals exhibited a reduction in force, fiber atrophy, and reduced myogenin expression. The mechanism of this protective effect is the avoidance of muscle proteolysis, probably mediated by calpain. In a similar study with rabbits, Sassoon et al40 showed that very high doses of methylprednisolone (60 mg/kg/day for 2 days) have no additive effects on diaphragmatic dysfunction induced by passive ACV. The same doses administered during ACV, however, produced a significant decrease in the maximal tetanic force elicited by the diaphragm.40 Thus, the effects of high-dose methylprednisolone on diaphragmatic function depend on the mode of ventilation: if the muscle contracts, the effects are injurious, whereas if the muscle is passive, the effects are protective or neutral.
Important data have appeared in the last few years regarding the effects of ACV in human subjects. Levine et al41 showed that complete diaphragmatic inactivity for 18 to 69 hours (mean: 34 hours) in brain-dead subjects resulted in marked atrophy of slow and fast-twitch fibers of the diaphragm as compared to matched controls (individuals who were ventilated for a scheduled surgery for 2 to 3 hours). The major mechanism explaining the diaphragmatic atrophy was increased muscle proteolysis. Peripheral skeletal muscles (pectoralis major) did not show histologic findings of atrophy. A subsequent study by Hussain et al,42 conducted in humans and using a similar design, extended the findings and provided data suggesting that both protein synthesis and breakdown are involved in the diaphragmatic dysfunction.
Jaber et al43 have investigated the time course of the decrease in diaphragm contractility in humans under passive ACV. The authors observed a progressive loss in diaphragmatic force, as reflected by measurements of tracheal pressure. The tracheal twitch pressure significantly decreased over time: mean reduction was 32% after 6 days of passive ventilation. The degree of muscle injury as detected by electron microscopy was significantly correlated with the duration of passive ACV. Again, upregulation of proteolytic systems played a major role in the ventilator-induced diaphragmatic injury induced by mechanical ventilation.
If passive ventilation is one extreme, the other is a fatiguing loading. Both extremes are harmful to the respiratory muscles. Normal subjects submitted to inspiratory-resistive loading up to a fatiguing threshold showed a decrease in diaphragmatic contractility lasting for at least 24 hours.44 Jiang et al45 showed diaphragmatic injury and inflammation at 3 days after a 90-minute period of acute moderate and high inspiratory-resistive loading in rabbits. The same group46 subsequently reported a marked decrease in the force production of the diaphragm at 3 days after high inspiratory-resistive loading over the same time. Such stress also induces selective upregulation of a number of cytokines in the diaphragmatic fibers, and eventually may lead to systemic effects.47,48 Toumpanakis et al49 have further analyzed the effects of inspiratory resistive breathing in rat lungs. The animals received 100% oxygen during the experiments. The authors showed that after 3 to 6 hours of stressful breathing, the alveolar–capillary membrane permeability increased, the static lung compliance decreased, and significant lung inflammation developed, as manifested by changes in histology (appearance of interstitial and intraalveolar neutrophils) and cytokine expression (increase in tumor necrosis factor and interleukin levels in lung tissue).
Research studies conducted in patients admitted to an ICU reveal that patients experience major sleep disturbances in terms of quantity and quality.50–52 The acuity of illness, the use of medications (such as sedatives or opioids), caregiver interventions, and environmental elements are contributing factors.50 Gabor et al53 indicated that only 30% of sleep disruption in ventilated patients was attributable to elements of the ICU environment.
Parthasarathy and Tobin54 sought to determine if sleep quality was influenced by the mode of ventilation. They hypothesized that sleep is more fragmented during pressure-support ventilation (PSV) as compared to ACV because of the development of central apneas. Eleven patients were ventilated with ACV at tidal volumes of 8 mL/kg, inspiratory flow rate 1 L/s, and a backup rate of four breaths below the total assisted rate. PSV was set to deliver the same tidal volume. Patients also received PSV with 100 mL of added dead space. During wakefulness, respiratory rate was similar with the two modes. During sleep, minute ventilation fell more during PSV than during ACV. Sleep fragmentation, measured as the number of arousals and awakenings, was significantly greater during PSV than during ACV (seventy-nine versus fifty-four events per hour). Six patients had apneas while receiving PSV, whereas none had apneas while receiving ACV. The percentage of patients who had congestive heart failure was significantly higher among patients exhibiting apneas than among patients free of apneas (83% vs. 20%). Minute ventilation during sleep was greater in patients who did not develop apneas, suggesting that increased drive protects against the development of apneas. The addition of dead space reduced the number of apneas markedly: from fifty-four to four apneas per hour. These data suggest that settings that generate overassistance promote the occurrence of apneas during assisted ventilation.
Cabello et al55 conducted a study in fifteen ventilator-dependent patients and compared three modes: ACV, PSV, and automatically adjusted PSV. The hypothesis was that PSV settings adjusted to patient ventilatory needs could improve sleep quality as compared to ACV. During ACV, settings were adjusted to provide a tidal volume of 8 mL/kg with a constant inspiratory flow of 1 L/s (and backup rate at 10 breaths/min). In the second arm, PSV was adjusted by clinicians to obtain a tidal volume of 6 to 8 mL/kg and a respiratory rate below 35 breaths/min. In the third arm, PSV was automatically regulated in a way that continuously adjusted the level of support so as to keep the patients within a comfort zone.56 PEEP was kept constant at 5 cm H2O, and patients were free of sedative drugs. The median tidal volumes (390 to 500 mL), respiratory rates (20 to 21 breaths/min), and minute ventilation did not differ between the three modes. Nine patients exhibited sleep apneas, and ten displayed ineffective efforts. The number of ineffective efforts per hour of sleep did not differ among the modes (mean: six to sixteen ineffective efforts per hour). The number of apneas was similar between the two PSV modalities (five to seven apneas per hour of sleep). Sleep fragmentation (arousals and awakenings per hour), sleep architecture, and sleep quantity did not differ among the modes. One explanation for the difference with the findings in the study by Parthasarathy and Tobin is that tidal volumes and minute ventilation were similar for all modes in the study of Cabello et al. The relative infrequency of ineffective efforts and apneas in this study suggests that patients were not overassisted. Together these data indicate that excessive ventilator support is central in the development of sleep fragmentation.
The clinical consequences of these sleep abnormalities are not known. Researchers have noted that sleep deprivation may generate immune suppression, loss of circadian hormonal secretion (melatonin and cortisol), profoundly alter respiratory muscles endurance and neurocognitive function, and modify the normal physiologic responses to hypoxia and hypercapnia.51,52 Whether the sleep disturbances are a marker of brain dysfunction related to critical illness or represent a specific syndrome with an independent effect on outcomes is not known.
Rationale, Advantages, and Limitations
The main reasons for using ACV are to unload the inspiratory muscles and to improve gas exchange. ACV permits complete respiratory muscle rest, which is usually the case when patients do not trigger the machine, and a variable degree of respiratory muscle work. ACV commonly achieves an improvement in gas exchange, and only a minority of ventilated patients die because of refractory hypoxemia.
During passive ventilation with ACV at a constant inspiratory flow, fundamental variables related to respiratory system mechanics, such as tidal volume, inspiratory flow, peak airway pressure, end-inspiratory plateau airway pressure, and total PEEP (the sum of external PEEP and intrinsic PEEP, if any), are measured easily (Fig. 6-2). These variables allow calculation of resistance, compliance, and the time constant of the respiratory system.
Figure 6-2
(From top to bottom) Tracings of airflow (FLOW), airway pressure (Paw), and tidal volume (VOLUME). Each mark on the time axis denotes 1 second. Note that expiratory flow is interrupted by the beginning of each breath, thus heralding dynamic hyperinflation. A prolonged end-inspiratory occlusion (fourth breath from the left) enables measurement of the static recoil pressure of the respiratory system. A prolonged end-expiratory occlusion (sixth breath from the left) illustrates the presence of intrinsic PEEP (3 cm H2O). These values, together with peak airway pressure, inspiratory flow rate, and tidal volume, enable the calculation of resistance, compliance, and respiratory system time constant in passively ventilated patients.
If airway pressure tracings are obtained during passive ACV as well as during patient-triggered ACV at the same settings, we can estimate a patient’s work of breathing simply by superimposing the two tracings (Fig. 6-3). When patients are triggering the breaths, the end-inspiratory plateau pressure also can be influenced by the amount and duration of inspiratory muscle effort (Fig. 6-4). When mechanical breaths are triggered by the patient, the scooping on the airway pressure profile allows indirect evaluation of patient–ventilator interaction (Fig. 6-5). Such capabilities are unique to ACV. These capabilities represent a major advantage because they enable one to properly understand respiratory system mechanics and patient–ventilator interactions.
Figure 6-3
(From top to bottom) Tracings of airflow (FLOW), airway pressure (Paw), esophageal pressure (Pes), and tidal volume (VOL). Each mark on the time axis denotes 1 second. The tracings in each panel were obtained from the same individual at two different times. They were then superimposed. Ventilator settings were identical. The vertical line on the airflow, airway pressure, and esophageal pressure tracings indicates the end of ventilator’s total inspiratory time. The dotted areas within the airway pressure and esophageal pressure tracings are identical. The dotted areas denote the amount of inspiratory muscle effort that the patient made during the assisted breath.
Figure 6-4
(From top to bottom) Tracings of airflow (FLOW), airway pressure (Paw), transdiaphragmatic pressure (Pdi), gastric pressure (Pga), and tidal volume (VOLUME). Each mark on the time axis denotes 1 second. The time of inspiratory flow is shorter than the duration of diaphragmatic contraction. This patient did not exhibit expiratory muscle recruitment, as indicated by the gastric pressure recording. Inspiratory muscles relax at the end of the inspiratory pause time, thus explaining the “M” wave shape on the airway pressure recording.
Figure 6-5
(From top to bottom) Tracings of airflow (FLOW), airway pressure (Paw), esophageal pressure (Pes), and tidal volume (VOLUME). Each mark on the time axis denotes 1 second. As seen easily on the esophageal pressure tracing, there is a highly variable inspiratory muscle effort over time, thus inducing permanent scooping on the airway pressure recording.
A major limitation of ACV is that it imposes a number of constraints on the variability of the patient’s breathing pattern: inspiratory flow, inspiratory time, and backup rate. Adjusting ACV settings may be more complex than with pressure-limited modes. One reason is that manufacturers employ different algorithms for implementing the delivery of a tidal breath. The other reason is that during ACV it is difficult to pinpoint the inspiratory flow rate and tidal volume settings that are optimal for an individual patient. Some settings are almost impossible to achieve with ACV. For instance, the simultaneous adjustment of a moderate tidal volume at a high inspiratory flow rate will produce a short machine inspiratory time, which, under certain circumstances, may not match the patient’s neural inspiratory time properly. In addition, the patient’s varying ventilatory needs and the change in the mechanical properties of the respiratory system over the course of ventilation imply that periods of underassist are likely to be interspersed with periods of overassist (Fig. 6-6). These problems, however, are common to most ventilator modes.
Figure 6-6
(From top to bottom) Tracings of airflow (FLOW), airway pressure (Paw), esophageal pressure (Pes), and tidal volume (VOLUME). Each mark on the time axis denotes 1 second. Note the marked breath-by-breath variability in inspiratory muscle effort (esophageal pressure swings) and airway pressure profile. There is profound patient–ventilator dyssynchrony, and numerous inspiratory attempts fail to trigger the ventilator. It is also remarkable how difficult it can be to estimate the end-inspiratory plateau airway pressure in such circumstances.
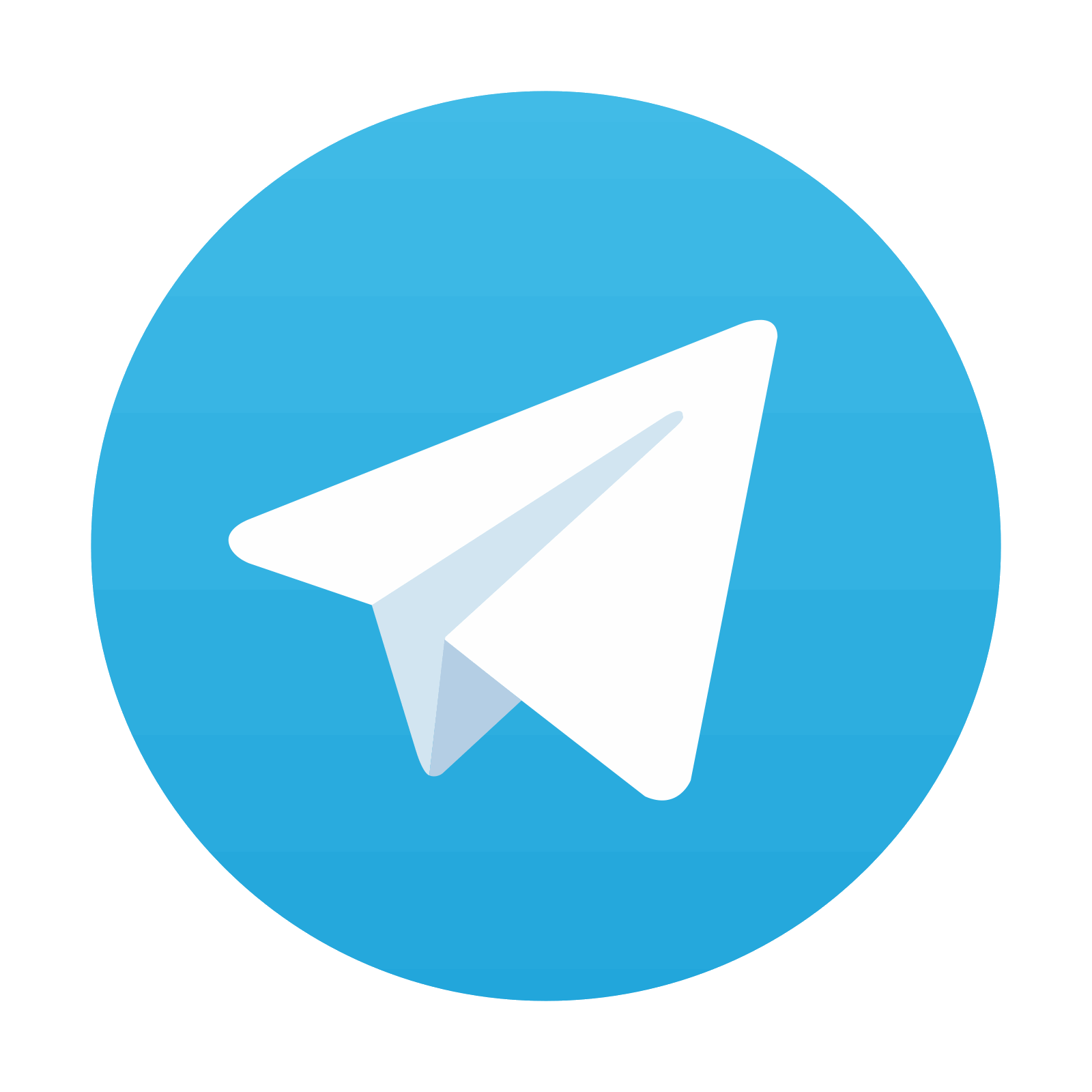
Stay updated, free articles. Join our Telegram channel
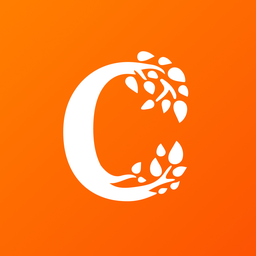
Full access? Get Clinical Tree
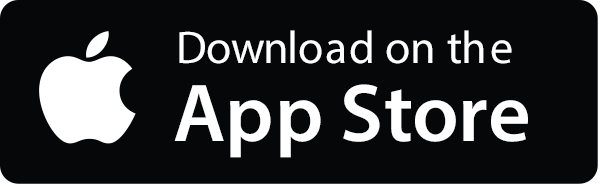
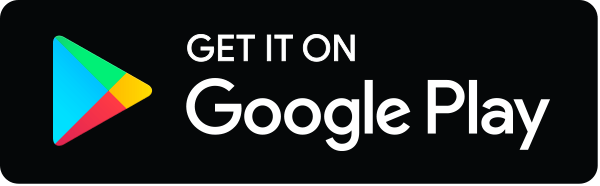