11 PHYSIOLOGIC BASIS OF MECHANICAL VENTILATION Respiratory Mechanics, Chest Wall Elastance, and Lung Volume Severity of Lung Injury and Lung Recruitability MECHANICAL VENTILATION IN ACUTE RESPIRATORY DISTRESS SYNDROME: AVAILABLE EVIDENCE INDIVIDUALIZING MECHANICAL VENTILATION IN PATIENTS WITH ACUTE RESPIRATORY DISTRESS SYNDROME POSSIBLE ADJUNCTS TO MECHANICAL VENTILATION Since the first description of acute respiratory distress syndrome (ARDS) in 1967,1 mechanical ventilation has been the primary “buying time” treatment for acute lung injury (ALI). Mechanical ventilation is not a “gas exchanger,” but instead replaces, totally or partially, the force normally generated by the respiratory muscles to promote ventilation, providing muscle rest. The effects of mechanical ventilation on gas exchange are indirect and include (1) better clearance of carbon dioxide (CO2) by the power of the mechanical ventilator to expand the diseased and collapsed lung; (2) improvement in oxygenation by preventing alveolar hypoxia caused by hypoventilation; (3) improvement in oxygenation by increasing inspiratory oxygen fraction, which affects alveolar partial pressure of oxygen (PAO2); (4) improvement in oxygenation by opening lung regions otherwise collapsed (alveolar recruitment); and (5) improving oxygenation by maintaining positive end-expiratory pressure (PEEP), preventing the collapse of the lung regions previously recruited during the inspiratory phase. Extensive reviews on the history of mechanical ventilation of ARDS can be found elsewhere.2,3 In the 1970s, mechanical ventilation was recommended and performed with low PEEP and high tidal volume: “. . . larger tidal volumes (10 to 15 per kilogram) are preferable, having been used in several thousand ventilated patients with no evidence of development of pulmonary damage.”4 Today, this advice seems inconceivable. At that time, the main concerns were the putative harm of high inspiratory oxygen fraction and the hemodynamic impairment. It was later recognized in experimental and clinical settings5–7 that high-volume/high-pressure mechanical ventilation could severely damage the lung parenchyma. Such lesions, primarily attributed to the excessive airway pressure, were collectively classified as barotrauma. In the same period, Suter and colleagues8 published a report that, for the first time, systematically described the interaction between PEEP, lung mechanics, gas exchange, and hemodynamics. In the 1980s, based on the work of Dreyfuss,9,10 the focus progressively shifted from the potential harm of pressure to the harm of volume (overdistention), a concept that was popularized as volutrauma.11 In the mid-1980s, the application of computed tomography (CT)12,13 and the quantitative approach to CT analysis14 led to the concept of baby lung,15,16 which accounted for most of the previous observations on respiratory mechanics, gas exchange functionality, and potential harm of mechanical ventilation. The premise of this line of thought is that high pressure or excessive distention applied to a small fraction of the lung parenchyma (with a size similar to the lung of a baby) unavoidably leads to structural lesions of the lung regions open to ventilation. Total lung rest was achievable with the use of extracorporeal CO2 removal, targeted to prevent the damages of high-pressure/high-volume ventilation.17–19 The target of mechanical ventilation shifted toward lung protection, rather than normal gas exchange functionality. Hickling and associates20 proposed the “permissive hypercapnia” strategy for ARDS, providing “gentle treatment” of the portion of the lung that remains open to ventilation (the baby lung) through less aggressive mechanical ventilation, at the acceptable price of an abnormally higher arterial partial pressure of carbon dioxide (PaCO2). A large amount of experimental and clinical data over the years has supported the approach of a lung protective strategy.21–25 Low tidal volume prevents the excessive global stress and strain of the baby lung, whereas higher PEEP prevents the regional excessive stress and strain by avoiding alveolar collapse and reopening during mechanical ventilation.26 These mechanical events are associated with an inflammatory reaction of the epithelial and endothelial lung cells (biotrauma/atelectrauma) as shown by the seminal study of Tremblay and colleagues27 (see also work of Dreyfuss and Saumon11 and Tremblay and Slutsky28). The literature to date supports the thought that the harm of mechanical ventilation is due to excessive global or regional stress and strain.29 This situation leads to two results—a physical rupture of the lung and a mechanically induced inflammation of the lung parenchyma, constituting VILI. The best “mechanical ventilation” would provide adequate gas exchange with the lowest amount of VILI. To ventilate the respiratory system, force is required. The driving force for ventilation under normal circumstances is provided by the respiratory muscles. During spontaneous breathing, the thoracic cage expands. This causes a decrease in intrathoracic pressure (pleural pressure, Ppl) relative to the atmosphere (in normal conditions approximately 2 mm Hg of ΔPpl is sufficient to expand the thoracic cage by 0.5 L). Because the lungs are connected in series with the thoracic cage, their volume is expanded to a near equal extent (not considering the blood shift30). The force that distends the lung is the pressure difference between the alveoli and the pleural cavity (the transpulmonary pressure, PL). As the lung expands, the alveolar pressure becomes subatmospheric, and gas flow is generated (inspiration). When the respiratory muscles relax, the potential energy accumulated in the respiratory system (lung and chest wall) returns the chest wall and the lung parenchyma to the resting position (expiration). In spontaneously breathing subjects, the driving force (muscular pressure, ΔPmusc) is spent partly to expand the chest wall (ΔPpl), partly to expand the lung (ΔPL), and partly to overcome the resistances to the gas flow. In quasi-static conditions, in which the resistances to gas flow are negligible: The transpulmonary pressure for a given driving pressure uniquely depends on the ratio of lung elastance to respiratory system elastance. In normal subjects EL/Etot is approximately 0.5, whereas in patients with ARDS it may range from 0.2 (e.g., in obese patients or in patients with high intra-abdominal pressure) to 0.8 (e.g., in patients with a very small baby lung and a normal chest wall elastance). This variability implies that for the same driving force applied and read on the ventilator display (e.g., 30 cm H2O), the resulting transpulmonary pressure may range from 6 to 24 cm H2O (Fig. 11.1). The transpulmonary pressure is applied to the force-bearing structure of lung parenchyma, the extracellular matrix, which constitutes the lung skeleton.31 The lung skeleton is a complex and metabolically active structure that includes a network of several components—elastin, collagen, and proteoglycans. All these molecules are involved in determining the mechanical characteristics of the respiratory system. The elastin may be considered as an elastic spring, whereas the unextensible collagen, which is folded at end expiration and completely unfolded at a lung volume equal to total lung capacity, acts as a stop-length fiber.32,33 The proteoglycans stabilize the collagen-elastin network, contributing to lung elasticity and alveolar stability at low and medium lung volumes.34 The matrix of elastin, collagen, and proteoglycans is arranged in two main fiber systems: (1) the axial system, which originates from the pulmonary hilum and runs deeply into the lung parenchyma down to the alveolar level, where it joins (2) the peripheral system, which originates from the visceral pleura and runs centripetally within the lung parenchyma.31 The lung skeleton may be considered as a continuous elastic structure that reaches its extension limits at total lung capacity, a lung volume equal to about threefold the lung resting volume. At this level of alveolar distention, the collagen is completely unfolded, and further expansion is prevented. The epithelial and endothelial cells do not directly bear the applied forces because they are anchored to the extracellular matrix by a series of structural proteins (integrins), which are connected to the cytoskeleton. During lung expansion, the epithelial and the endothelial cells modify their shape. It is well documented that mechanically induced cellular deformation activates a series of mechanosensors with the production of several inflammatory mediators, such as cytokines (interleukin 6, tumor necrosis factor-α, and interferon-γ),27,35 metalloproteinases (enzymes involved in the remodeling of the matrix),36 leukotrienes,37 and interleukin 8,38–40 the most powerful attractor of neutrophils.41 Gross barotrauma (e.g., pneumothorax) is due to the stress at rupture of the lung skeleton, whereas intrapulmonary inflammation is primarily due to the excessive strain of the epithelial and endothelial cells. where ΔV is the volume variation applied to the lung (i.e., the tidal volume), and V0 is the lung resting volume (i.e., the functional residual capacity at atmospheric pressure [without any application of PEEP]). The lung-specific elastance is the transpulmonary pressure required to double the lung resting volume (i.e., the ΔPL when ΔV/V0 is equal to 1). In ARDS, lung-specific elastance is similar to normal,16,42 reinforcing the concept of the baby lung (lung is small and not stiff), and questions the use of normalizing the tidal volume to the ideal body weight. The same tidal volume per kilogram may result in completely different strain according to the size of the baby lung (the V0 of the previous equation). For example, a 70-kg man with ARDS may have, according to the severity of the lung injury, a residual baby lung equal to 60%, 40%, or 20% of his normal lung size. If the ventilator is set to deliver 10 mL/kg, the actual delivered tidal volume would generate an alveolar strain, which would result from the application, in normal lung, of a tidal volume equal to 17 mL/kg, 25 mL/kg, and 50 mL/kg, values associated with a significant lung injury in laboratory studies.11,29 Recently we attempted to quantify the relationship between stress-strain and VILI in healthy animals. We found that edema formation was a threshold phenomenon, induced by mechanical ventilation when the global strain reaches a critical value of about 2.43 This threshold roughly corresponds to the point where the stress-strain curve loses its linearity and starts an exponential growth, indicating that some lung regions reach their own total capacity and cannot expand any further. At this level of strain, in a period of 24 to 48 hours the mechanical ventilation is lethal and the increased lung weight (two to three times the baseline) is associated with a striking impairment of respiratory mechanics, gas exchange, hemodynamics, inflammation, distal organs damage, and 100% mortality rate. This lethal strain, and associated stress, however, is rarely applied in clinical practice. To explain VILI in a diseased lung, therefore, alternative phenomena must be taken into account as the lung dishomogeneity and the presence of stress rise, which will be discussed later. PaO2, PaO2-to-fraction of inspired oxygen ratio (PaO2/FIO2), and Riley’s shunt fraction44 are the most commonly used variables to assess the severity of lung injury. In particular, the PaO2/FIO2 thresholds of 300 and 200 were used to define ALI (300) and ARDS (200) according to the American-European Consensus Conference on ARDS.45 Consequently, the PaO2/FIO2 ratio is perceived as a key variable by most intensive care unit (ICU) physicians: The lower the PaO2/FIO2 ratio, the greater the lung injury. This equivalence is highly questionable. First, in most large studies on ARDS, no association was found between hypoxemia and outcome.46 In the large study showing a better outcome with low tidal volume compared with higher tidal volume, the PaO2/FIO2 ratio was significantly lower in the low tidal volume group despite ending with better outcome.25 Finally, the PaO2/FIO2 ratio was not different in patients with early, intermediate, and late ARDS, suggesting that oxygenation was not dependent on the structural changes of lung parenchyma occurring with time.47 In a study48 in which the lung severity was assessed by CT scan (and defined as a fraction of the gasless tissue), we did not find significant changes of PaO2/FIO2 ratio over a wide range of nonaerated tissue (Fig. 11.2), and PaO2/FIO2 ratio was not associated with outcome. Most data suggest that PaO2/FIO2 ratio is a weak indicator of the overall lung severity, with compensatory rearrangement of perfusion during ARDS limiting the deterioration of oxygenation. The same limits apply when PaO2/FIO2 ratio changes are used to assess lung recruitment. Because this maneuver is unavoidably associated with changes of perfusion (global or regional), the increase of PaO2/FIO2 ratio may be partly due to decrease of perfusion, as shown in the 1980s.49–51 Most data suggest that the use of oxygenation variables alone to assess lung severity is misleading. Although less considered, the variables derived from CO2, as the total or alveolar deadspace, seem to be of greater value in assessing lung severity. It has been shown in ALI/ARDS patients that deadspace at entry is a strong predictor of outcome,52 and that PaCO2 for the same total ventilation steadily increases from early to intermediate and to late ARDS, reflecting the lung structural changes.47 The PCO2 response to prone position (in contrast to PO2 response) is a strong prognostic index of mortality.53 Most data suggest that CO2-related variables (deadspace), more than PaO2/FIO2 ratio, reflect the severity of lung injury (and associated mortality rate) at the time of presentation and the structural changes of lung parenchyma occurring with time (fibrosis, Pneumocystis, and possibly perfusion defects). In the original description of ARDS,1 the low compliance (i.e., high elastance) of the respiratory system was a landmark of the syndrome. The respiratory system compliance is not considered in the current definition of ARDS, however.45 For years, the low compliance was attributed uniquely to the lung component (lung stiffness and lack of surfactant). Quantitative CT shows, however, that the respiratory system compliance primarily reflects the size of lung open to gases (the baby lung14,16), suggesting that the intrinsic functioning lung elasticity in ARDS is close to normal (the lung is “small” rather than “stiff”). More than gas exchange, the respiratory system compliance indirectly assesses the lung injury severity (the smaller baby lung, the greater lung injury),54 as confirmed in animal experiments.55 Another variable also must be taken into account—the elastance of the chest wall. It has been shown in a significant portion of ALI/ARDS patients that the chest wall elastance is greater than normal because of increased intra-abdominal pressure, obesity, or severe edema.56 In patients with extrapulmonary ARDS57 and in obese patients,58 the high elastance of the respiratory system may be due to the chest wall and to the lung derangement. Measurement of intra-abdominal pressure should be considered when selecting mechanical ventilator settings. CT can be used to assess the severity of lung injury by measuring the fraction of nonaerated lung tissue at end expiration (end expiration pause at 5 cm H2O). This fraction includes the lung tissue that is “consolidated” (i.e., not openable at 45 cm H2O airway pressure) and the tissue that is collapsed but openable at 45 cm H2O. These values were chosen to produce a minimal risk during the maneuver and because this is the most frequently used in the literature for recruitment maneuver.59 In patients with elevated chest wall elastance, the resulting transpulmonary pressure could be insufficient, however, to overcome the opening pressure of some pulmonary units. The total fractions of nonaerated lung tissue (consolidated plus recruitable) and the recruitable tissue alone (tissue that regains aeration at 45 cm H2O airway pressure) are strongly associated with mortality rate. The data of Figure 11.2 show the inadequacy of the term ALI/ARDS, as currently defined, to describe the lung injury.48 The data refer to a population of ALI/ARDS patients, classified according to the American-European Consensus Conference on ARDS,45 in which a CT-based quantitative analysis of the whole lung parenchyma at 5 cm H2O PEEP was performed. As shown in Figure 11.3, the fraction of the nonaerated lung tissue (consolidated or collapsed or both) may range from 5% to 70% of the entire lung parenchyma. Patients meeting ALI/ARDS criteria may have a baby lung size very close to that of normal subjects, or a baby lung that is just a small fraction of the expected normal lung. When the distending force is applied to the lungs by the mechanical ventilator, previously collapsed lung regions may open. Lung recruitability may be expressed as the amount of lung tissue regaining aeration when increasing the applied driving force from 5 to 45 cm H2O. As shown in Figure 11.3, in some patients, lung recruitability was almost negligible, whereas in others it was equal to 25% to 35% of the entire lung parenchyma. Lung recruitability was strongly associated with the fraction of nonaerated tissue, suggesting that the greater the inflammatory edema, the greater the lung collapse. It seems that the best way to assess the overall lung severity and the related lung recruitability, both strongly associated with mortality rate, is the CT scan analysis. Physiologic variables are poor indicators of the severity of the lung injury. This relationship is shown in Figure 11.4—for a large variation of lung injury, ranging from 20% to 60% of the lung parenchyma, the values of PaO2/FIO2 ratio, lung compliance, and PaCO2 greatly overlap. Figure 11.4 Mean ± standard deviation values of respiratory variables of a population of 68 patients with acute lung injury/acute respiratory distress syndrome. Blue columns represent PaO2/FIO2 ratio at positive end-expiratory pressure (PEEP) 5 cm H2O (mm Hg), red columns represent respiratory system compliance at PEEP 5 cm H2O (mL/cm H2O), green columns represent deadspace fraction (%), and gold columns represent alveolar deadspace fraction (%). *P < 0.05 versus patients with a fraction of nonaerated tissue recorded at 5 cm H2O PEEP less than 0.2. †P < 0.05 versus patients with a fraction of nonaerated tissue recorded at 5 cm H2O PEEP ranging from 0.2 to 0.4. The continuous purple line represents the likelihood of death predicted by the fraction of nonaerated lung tissue recorded at 5 cm H2O PEEP (P = 0.015). Weight is a poor surrogate of strain because ARDS patients with similar body weights may have completely different lung sizes and consequently different levels of strain at equal tidal volumes. This is confirmed by the plateau pressures measured in these different studies (see Fig. 11.5). As shown for the same tidal volume per ideal body weight, the plateau pressures are widely distributed with huge overlap between the studies. This reflects a wide distribution of the respiratory system compliance and consequently of the lung size. Although, in our opinion, the definition of ARDS should include a quantitative estimate of the lung edema, because this is not feasible in most of the ICU, alternative ways for defining ARDS have been sought. ARDS was first defined by Ashbaugh and coworkers in 1967.1
Mechanical Ventilation in Acute Respiratory Distress Syndrome
History
Physiologic Basis of Mechanical Ventilation
Driving Force
Transpulmonary Pressure
Force-Bearing Structure of Lung Parenchyma
Concept of Stress and Strain
Patient Characterization
Gas Exchange
Oxygen
Carbon Dioxide
Respiratory Mechanics, Chest Wall Elastance, and Lung Volume
Severity of Lung Injury and Lung Recruitability
Ards Classification
Stay updated, free articles. Join our Telegram channel
Full access? Get Clinical Tree
Mechanical Ventilation in Acute Respiratory Distress Syndrome
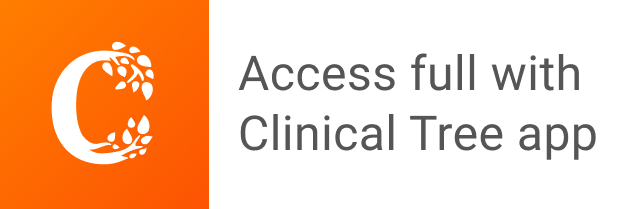