Key Concepts
Osmotic pressure is generally dependent only on the number of nondiffusible solute particles. This is because the average kinetic energy of particles in solution is similar regardless of their mass.
Potassium is the most important determinant of intracellular osmotic pressure, whereas sodium is the most important determinant of extracellular osmotic pressure.
Fluid exchange between the intracellular and interstitial spaces is governed by the osmotic forces created by differences in nondiffusible solute concentrations.
Serious manifestations of hyponatremia are generally associated with plasma sodium concentrations <120 mEq/L.
Very rapid correction of hyponatremia has been associated with demyelinating lesions in the pons (central pontine myelinolysis), resulting in serious permanent neurological sequelae.
The major hazard of increases in extracellular volume is impaired gas exchange due to pulmonary interstitial edema, alveolar edema, or large collections of pleural or ascitic fluid.
Intravenous replacement of potassium chloride is usually reserved for patients with, or at risk for, significant cardiac manifestations or severe muscle weakness.
Because of its lethal potential, hyperkalemia exceeding 6 mEq/L should always be corrected.
Symptomatic hypercalcemia requires rapid treatment. The most effective initial treatment is rehydration followed by a brisk diuresis (urinary output 200-300 mL/h) utilizing administration of intravenous saline infusion and a loop diuretic to accelerate calcium excretion.
Symptomatic hypocalcemia is a medical emergency and should be treated immediately with intravenous calcium chloride (3-5 mL of a 10% solution) or calcium gluconate (10-20 mL of a 10% solution).
Some patients with severe hypophosphatemia may require mechanical ventilation postoperatively because of muscle weakness.
Marked hypermagnesemia can lead to respiratory and cardiac arrest.
Isolated hypomagnesemia should be corrected prior to elective procedures because of its potential for causing cardiac arrhythmias.
Management of Patients with Fluid & Electrolyte Disturbances: Introduction
Fluid and electrolyte disturbances are extremely common in the perioperative period. Large volumes of intravenous fluids are frequently required to correct fluid deficits and compensate for blood loss during surgery. Major disturbances in fluid and electrolyte balance can rapidly alter cardiovascular, neurological, and neuromuscular functions, and anesthesia providers must have a clear understanding of normal water and electrolyte physiology. This chapter examines the body’s fluid compartments and common water and electrolyte derangements, their treatment, and anesthetic implications. Acid-base disorders and intravenous fluid therapy are discussed in other chapters.
Nomenclature of Solutions
The system of international units (SI) has still not gained universal acceptance in clinical practice, and many older expressions of concentration remain in common use. Thus, for example, the quantity of a solute in a solution may be expressed in grams, moles, or equivalents. To complicate matters further, the concentration of a solution may be expressed either as quantity of solute per volume of solution or quantity of solute per weight of solvent.
One mole of a substance represents 6.02 × 1023 molecules. The weight of this quantity in grams is commonly referred to as gram-molecular weight. Molarity is the standard SI unit of concentration that expresses the number of moles of solute per liter of solution. Molality is an alternative term that expresses moles of solute per kilogram of solvent. Equivalency is also commonly used for substances that ionize: the number of equivalents of an ion in solution is the number of moles multiplied by its charge (valence). Thus, a 1 M solution of MgCl2 yields 2 equivalents of magnesium per liter and 2 equivalents of chloride per liter.
Osmosis is the net movement of water across a semipermeable membrane as a result of a difference in nondiffusible solute concentrations between the two sides. Osmotic pressure is the pressure that must be applied to the side with more solute to prevent a net movement of water across the membrane to dilute the solute.
Osmotic pressure is generally dependent only on the number of nondiffusible solute particles. This is because the average kinetic energy of particles in solution is similar regardless of their mass. One osmole equals 1 mol of nondissociable substances. For substances that ionize, however, each mole results in n Osm, where n is the number of ionic species produced. Thus, 1 mol of a highly ionized substance such as NaCl dissolved in solution should produce 2 Osm; in reality ionic interaction between the cation and anion reduces the effective activity of each such that NaCl behaves as if it is only 75% ionized. A difference of 1 mOsm/L between two solutions results in an osmotic pressure of 19.3 mm Hg. The osmolarity of a solution is equal to the number of osmoles per liter of solution, whereas its osmolality equals the number of osmoles per kilogram of solvent. Tonicity, a term that is often used interchangeably with osmolarity and osmolality, refers to the effect a solution has on cell volume. An isotonic solution has no effect on cell volume, whereas hypotonic and hypertonic solutions increase and decrease cell volume, respectively.
Fluid Compartments
Body water is distributed between two major fluid compartments separated by cell membranes: intracellular fluid (ICF) and extracellular fluid (ECF). The latter can be further subdivided into intravascular and interstitial compartments. The interstitium includes all fluid that is both outside cells and outside the vascular endothelium. The relative contributions of each compartment to total body water (TBW) and body weight are delineated in Table 49-1.
Compartment | Fluid as Percent Body Weight (%) | Total Body Water (%) | Fluid Volume (L) |
---|---|---|---|
Intracellular | 40 | 67 | 28 |
Extracellular | |||
Interstitial | 15 | 25 | 10.5 |
Intravascular | 5 | 8 | 3.5 |
Total | 60 | 100 | 42 |
The volume of fluid (water) within a compartment is determined by its solute composition and concentrations (Table 49-2). Differences in solute concentrations are largely due to the characteristics of the physical barriers that separate compartments (see below). The osmotic forces created by “trapped” solutes govern the distribution of water between compartments and ultimately each compartment’s volume.
Extracellular | ||||
---|---|---|---|---|
Gram-Molecular Weight | Intracellular (mEq/L) | Intravascular (mEq/L) | Interstitial (mEq/L) | |
Sodium | 23.0 | 10 | 145 | 142 |
Potassium | 39.1 | 140 | 4 | 4 |
Calcium | 40.1 | <1 | 3 | 3 |
Magnesium | 24.3 | 50 | 2 | 2 |
Chloride | 35.5 | 4 | 105 | 110 |
Bicarbonate | 61.0 | 10 | 24 | 28 |
Phosphorus | 31.01 | 75 | 2 | 2 |
Protein (g/dL) | 16 | 7 | 2 |
The outer membrane of cells plays an important role in regulating intracellular volume and composition. A membrane-bound adenosine triphosphate (ATP)-dependent pump exchanges Na+ for K+ in a 3:2 ratio. Because cell membranes are relatively impermeable to sodium and (to a lesser extent) potassium ions, potassium is concentrated intracellularly, whereas sodium is concentrated extracellularly. As a result, potassium is the most important determinant of intracellular osmotic pressure, whereas sodium is the most important determinant of extracellular osmotic pressure.
The impermeability of cell membranes to most proteins results in a high intracellular protein concentration. Because proteins act as nondiffusible solutes (anions), the unequal exchange ratio of 3 Na+ for 2 K+ by the cell membrane pump is critical in preventing relative intracellular hyperosmolality. Interference with Na+-K+-ATPase activity, as occurs during ischemia or hypoxia, results in progressive swelling of cells.
The principal function of ECF is to provide a medium for delivery of cell nutrients and electrolytes and for removal of cellular waste products. Maintenance of a normal extracellular volume—particularly the circulating component (intravascular volume)—is critical. For the reasons described above, sodium is quantitatively the most important extracellular cation and the major determinant of extracellular osmotic pressure and volume. Changes in ECF volume are therefore related to changes in total body sodium content. The latter is a function of sodium intake, renal sodium excretion, and extrarenal sodium losses (see below).
Very little interstitial fluid is normally in the form of free fluid. Most interstitial water is in chemical association with extracellular proteoglycans, forming a gel. Interstitial fluid pressure is generally thought to be negative (about −5 mm Hg). As interstitial fluid volume increases, interstitial pressure also rises and eventually becomes positive. When the latter occurs, the free fluid in the gel increases rapidly and appears clinically as edema.
Because only small quantities of plasma proteins can normally cross capillary clefts, the protein content of interstitial fluid is relatively low (2 g/dL). Protein entering the interstitial space is returned to the vascular system via the lymphatic system.
Intravascular fluid, commonly referred to as plasma, is restricted to the intravascular space by the vascular endothelium. Most electrolytes (small ions) freely pass between plasma and the interstitium, resulting in nearly identical electrolyte composition. However, the tight intercellular junctions between adjacent endothelial cells impede the passage of plasma proteins to outside the intravascular compartment. As a result, plasma proteins (mainly albumin) are the only osmotically active solutes in fluid not normally exchanged between plasma and interstitial fluid.
Increases in extracellular volume are normally proportionately reflected in intravascular and interstitial volume. However, when interstitial pressure becomes positive, continued increases in ECF result in expansion of only the interstitial fluid compartment (Figure 49-1). In this way, the interstitial compartment acts as an overflow reservoir for the intravascular compartment. This is seen clinically in the form of tissue edema.
Diffusion is the random movement of molecules due to their kinetic energy and is responsible for the majority of fluid and solute exchange between compartments. The rate of diffusion of a substance across a membrane depends upon (1) the permeability of that substance through that membrane, (2) the concentration difference for that substance between the two sides, (3) the pressure difference between either side because pressure imparts greater kinetic energy, and (4) the electrical potential across the membrane for charged substances.
Diffusion between interstitial fluid and ICF may take place by one of several mechanisms: (1) directly through the lipid bilayer of the cell membrane, (2) through protein channels within the membrane, or (3) by reversible binding to a carrier protein that can traverse the membrane (facilitated diffusion). Oxygen, CO2, water, and lipid-soluble molecules penetrate the cell membrane directly. Cations such as Na+, K+, and Ca2+ penetrate the membrane poorly because of the cell transmembrane voltage potential (which is positive to the outside) created by the Na+-K+ pump. Therefore, these cations can diffuse only through specific protein channels. Passage through these channels is dependent on membrane voltage and the binding of ligands (such as acetylcholine) to the membrane receptors. Glucose and amino acids diffuse with the help of membrane-bound carrier proteins.
Fluid exchange between the intracellular and interstitial spaces is governed by the osmotic forces created by differences in nondiffusible solute concentrations. Relative changes in osmolality between the intracellular and interstitial compartments result in a net water movement from the hypoosmolar to the hyperosmolar compartment.
Capillary walls are typically 0.5 μm thick, consisting of a single layer of endothelial cells with their basement membrane. Intercellular clefts, 6-7 nm wide, separate each cell from its neighbors. Oxygen, CO2, water, and lipid-soluble substances can penetrate directly through both sides of the endothelial cell membrane. Only low-molecular-weight water-soluble substances such as sodium, chloride, potassium, and glucose readily cross intercellular clefts. High-molecular-weight substances such as plasma proteins penetrate the endothelial clefts poorly (except in the liver and the lungs, where the clefts are larger).
Fluid exchange across capillaries differs from that across cell membranes in that it is governed by significant differences in hydrostatic pressures in addition to osmotic forces (Figure 49-2). These forces are operative on both arterial and venous ends of capillaries, with a tendency for fluid to move out of capillaries at the arterial end and back into capillaries at the venous end. Moreover, the magnitude of these forces differs between the various tissue beds. Arterial capillary pressure is determined by precapillary sphincter tone. Thus capillaries that require a high pressure such as glomeruli have low precapillary sphincter tone, whereas the normally low-pressure capillaries of muscle have high precapillary sphincter tone. Normally, all but 10% of the fluid filtered is reabsorbed back into capillaries. What is not reabsorbed (about 2 mL/min) enters the interstitial fluid and is then returned by lymphatic flow to the intravascular compartment.
Disorders of Water Balance
The human body at birth is approximately 75% water by weight. By 1 month this value decreases to 65%, and by adulthood to 60% for males and 50% for females. The higher fat content in females decreases water content. For the same reason, obesity and advanced age further decrease water content.
The normal adult daily water intake averages 2500 mL, which includes approximately 300 mL as a byproduct of the metabolism of energy substrates. Daily water loss averages 2500 mL and is typically accounted for by 1500 mL in urine, 400 mL in respiratory tract evaporation, 400 mL in skin evaporation, 100 mL in sweat, and 100 mL in feces. Evaporative loss is very important in thermoregulation because this mechanism normally accounts for 20-25% of heat loss.
Both ICF and ECF osmolalities are tightly regulated to maintain normal water content in tissues. Changes in water content and cell volume can induce serious impairment of function, particularly in the brain (see below).
The osmolality of ECF is equal to the sum of the concentrations of all dissolved solutes. Because Na+ and its anions account for nearly 90% of these solutes, the following approximation is valid:
Plasma osmolality = 2 × Plasma sodium concentration
Moreover, because ICF and ECF are in osmotic equilibrium, plasma sodium concentration [Na+]plasma generally reflects total body osmolality:
Because sodium and potassium are the major intra- and extracellular solutes, respectively:
Combining the two approximations:
Using these principles, the effect of isotonic, hypotonic, and hypertonic fluid loads on compartmental water content and plasma osmolality can be calculated (Table 49-3). The potential importance of intracellular potassium concentration is readily apparent from this equation. Thus significant potassium losses may contribute to hyponatremia.
| ||
Intracellular | Extracellular | |
Osmolality | 280 | 280 |
Volume (L) | 25 | 17 |
Net water gain | 0 | 0 |
| ||
Intracellular | Extracellular | |
Osmolality | 280 | 280 |
Volume (L) | 25 | 19 |
Net water gain | 0 | 2 |
Net effect: Fluid remains in extracellular compartment. | ||
| ||
Intracellular | Extracellular | |
Osmolality | 267.0 | 267.0 |
Volume (L) | 26.2 | 17.8 |
Net water gain | +1.2 | +0.8 |
Net effect: Fluid distributes between both compartments. | ||
| ||
Intracellular | Extracellular | |
Osmolality | 294.0 | 294.0 |
Volume (L) | 23.8 | 18.2 |
Net water gain | -1.2 | +1.2 |
Net effect: An intracellular to extracellular movement of water. |
In pathological states, glucose and—to a much lesser extent—urea can contribute significantly to extracellular osmolality. A more accurate approximation of plasma osmolality is therefore given by the following equation:
where [Na+] is expressed as mEq/L and blood urea nitrogen (BUN) and glucose as mg/dL. Urea is an ineffective osmole because it readily permeates cell membranes and is therefore frequently omitted from this calculation:
Plasma osmolality normally varies between 280 and 290 mOsm/L. Plasma sodium concentration decreases approximately 1 mEq/L for every 62 mg/dL increase in glucose concentration. A discrepancy between the measured and calculated osmolality is referred to as an osmolal gap. Significant osmolal gaps indicate a high concentration of an abnormal osmotically active molecule in plasma such as ethanol, mannitol, methanol, ethylene glycol, or isopropyl alcohol. Osmolal gaps may also be seen in patients with chronic kidney failure (attributed to retention of small solutes), patients with ketoacidosis (as a result of a high concentration of ketone bodies), and those receiving large amounts of glycine (as during transurethral resection of the prostate). Lastly, osmolal gaps may also be present in patients with marked hyperlipidemia or hyperproteinemia. In such instances, the protein or lipid part of plasma contributes significantly to plasma volume; although plasma [Na+] is decreased, [Na+] in the water phase of plasma (true plasma osmolality) remains normal. The water phase of plasma is normally only 93% of its volume; the remaining 7% consists of plasma lipids and proteins.
Plasma osmolality is closely regulated by osmoreceptors in the hypothalamus. These specialized neurons control both the secretion of antidiuretic hormone (ADH) and the thirst mechanism. Plasma osmolality is therefore maintained within relatively narrow limits by varying both water intake and water excretion.
Specialized neurons in the supraoptic and paraventricular nuclei of the hypothalamus are very sensitive to changes in extracellular osmolality. When ECF osmolality increases, these cells shrink and release ADH from the posterior pituitary. ADH markedly increases water reabsorption in renal collecting tubules (see Chapter 29), which tends to reduce plasma osmolality back to normal. Conversely, a decrease in extracellular osmolality causes osmoreceptors to swell and suppresses the release of ADH. Decreased ADH secretion allows a water diuresis, which tends to increase osmolality to normal. Peak diuresis occurs once circulating ADH is metabolized (90-120 min). With complete suppression of ADH secretion, the kidneys can excrete up to 10-20 L of water per day.
The carotid baroreceptors and probably atrial stretch receptors can also stimulate ADH release following a 5-10% decrease in blood volume. Other nonosmotic stimuli include pain, emotional stress, and hypoxia.
Osmoreceptors in the lateral preoptic area of the hypothalamus are also very sensitive to changes in extracellular osmolality. Activation of these neurons by increases in ECF osmolality induces thirst and causes the individual to drink water. Conversely, hypoosmolality suppresses thirst. Thirst is the major defense mechanism against hyperosmolality and hypernatremia, because it is the only mechanism that increases water intake.
Hyperosmolality occurs whenever total body solute content increases relative to TBW and is usually, but not always, associated with hypernatremia ([Na+] > 145 mEq/L). Hyperosmolality without hypernatremia may be seen during marked hyperglycemia or following the accumulation of abnormal osmotically active substances in plasma (see above). In the latter two instances, plasma sodium concentration may actually decrease as water is drawn from the intracellular to the extracellular compartment. For every 100 mg/dL increase in plasma glucose concentration, plasma sodium decreases approximately 1.6 mEq/L.
Hypernatremia is nearly always the result of either a relative loss of water in excess of sodium (hypotonic fluid loss) or the retention of large quantities of sodium. Even when renal concentrating ability is impaired, thirst is normally highly effective in preventing hypernatremia. Hypernatremia is therefore most commonly seen in debilitated patients who are unable to drink, the very aged, the very young, and patients with altered consciousness. Patients with hypernatremia may have a low, normal, or high total body sodium content (Table 49-4).
|
Solute diuresis
|
Excessive water losses
|
Combined disorders
|
These patients have lost both sodium and water, but the water loss is in relative excess to that of the sodium loss. Hypotonic losses can be renal (osmotic diuresis) or extrarenal (diarrhea or sweat). In either case, patients usually manifest signs of hypovolemia (see Chapter 51). Urinary sodium concentration is generally greater than 20 mEq/L with renal losses and less than 10 mEq/L with extrarenal losses.
This group of patients generally manifests signs of water loss without overt hypovolemia unless the water loss is massive. Total body sodium content is generally normal. Nearly pure water losses can occur via the skin, respiratory tract, or kidneys. Occasionally transient hypernatremia is observed with movement of water into cells following exercise, seizures, or rhabdomyolysis. The most common cause of hypernatremia in conscious patients with normal total body sodium content is diabetes insipidus. Diabetes insipidus is characterized by marked impairment in renal concentrating ability that is due either to decreased ADH secretion (central diabetes insipidus) or failure of the renal tubules to respond normally to circulating ADH (nephrogenic diabetes insipidus). Rarely, “essential hypernatremia” may be encountered in patients with central nervous system disorders. These patients appear to have “reset” osmoreceptors that function at a higher baseline osmolality.
Lesions in or around the hypothalamus and the pituitary stalk frequently produce diabetes insipidus. Diabetes insipidus often develops with brain death. Transient diabetes insipidus is also commonly seen following neurosurgical procedures and head trauma. The diagnosis is suggested by a history of polydipsia, polyuria (often >6 L/d), and the absence of hyperglycemia. In the perioperative setting, the diagnosis of diabetes insipidus is suggested by marked polyuria without glycosuria and a urinary osmolality lower than plasma osmolality. The absence of thirst in unconscious individuals leads to marked water losses and can rapidly produce hypovolemia. The diagnosis of central diabetes insipidus is confirmed by an increase in urinary osmolality following the administration of exogenous ADH. Aqueous vasopressin (5-10 units subcutaneously or intramuscularly every 4-6 h) is the treatment of choice for acute central diabetes insipidus. Vasopressin in oil (0.3 mL intramuscularly every day) is longer lasting but is more likely to cause water intoxication. Desmopressin (DDAVP), a synthetic analogue of ADH with a 12- to 24-h duration of action, is available as an intranasal preparation (10-40 mcg/d either as a single daily dose or divided into two doses) that can be used in both ambulatory and perioperative settings.
Nephrogenic diabetes insipidus can be congenital but is more commonly secondary to other disorders, including chronic kidney disease, hypokalemia and hypercalcemia, sickle cell disease, and hyperproteinemias. Nephrogenic diabetes insipidus can also be secondary to the side effects of some drugs (amphotericin B, lithium, demeclocycline, ifosfamide, mannitol). ADH secretion in nephrogenic diabetes insipidus is normal, but the kidneys fail to respond to ADH; urinary concentrating ability is therefore impaired. The mechanism may be either a decreased response to circulating ADH or interference with the renal countercurrent mechanism. The diagnosis is confirmed by failure of the kidneys to produce hypertonic urine following the administration of exogenous ADH. Treatment is generally directed at the underlying illness and ensuring an adequate fluid intake. Volume depletion by a thiazide diuretic can paradoxically decrease urinary output by reducing water delivery to collecting tubules. Sodium and protein restriction can similarly reduce urinary output.
This condition most commonly results from the administration of large quantities of hypertonic saline solutions (3% NaCl or 7.5% NaHCO3). Patients with primary hyperaldosteronism and Cushing’s syndrome may also have elevations in serum sodium concentration along with signs of increased sodium retention.
Neurological manifestations predominate in patients with hypernatremia and are generally thought to result from cellular dehydration. Restlessness, lethargy, and hyperreflexia can progress to seizures, coma, and ultimately death. Symptoms correlate more closely with the rate of movement of water out of brain cells than with the absolute level of hypernatremia. Rapid decreases in brain volume can rupture cerebral veins and result in focal intracerebral or subarachnoid hemorrhage. Seizures and serious neurological damage are common, particularly in children with acute hypernatremia when plasma [Na+] exceeds 158 mEq/L. Chronic hypernatremia is usually better tolerated than the acute form. After 24-48 h, intracellular osmolality begins to rise as a result of increases in intracellular inositol and amino acid (glutamine and taurine) concentrations. As intracellular solute concentration increases, neuronal water content slowly returns to normal.
The treatment of hypernatremia is aimed at restoring plasma osmolality to normal as well as correcting the underlying cause. Water deficits should generally be corrected over 48 h with a hypotonic solution such as 5% dextrose in water (see below). Abnormalities in extracellular volume must also be corrected (Figure 49-3). Hypernatremic patients with decreased total body sodium should be given isotonic fluids to restore plasma volume to normal prior to treatment with a hypotonic solution. Hypernatremic patients with increased total body sodium should be treated with a loop diuretic along with intravenous 5% dextrose in water. The treatment of diabetes insipidus is discussed above.
Rapid correction of hypernatremia can result in seizures, brain edema, permanent neurological damage, and even death. Serial Na+ osmolalities should be obtained during treatment. In general, decreases in plasma sodium concentration should not proceed at a rate faster than 0.5 mEq/L/h.
A 70-kg man is found to have a plasma [Na+] of 160 mEq/L. What is his water deficit?
If one assumes that hypernatremia in this cases represents water loss only, then total body osmoles are unchanged. Thus, assuming a normal [Na+] of 140 mEq/L and TBW content that is 60% of body weight:
Normal TBW × 140 = present TBW × [Na+]plasma or (70 × 0.6) × 140 = present TBW × 160
Solving the equation:
Present TBW = 36.7 L
Water deficit = normal TBW – present TBW or (70 × 0.6) – 36.7 = 5.3 L
To replace this deficit over 48 h, it is necessary to give 5% dextrose in water intravenously, 5300 mL over 48 h, or 110 mL/h.
Note that this method ignores any coexisting isotonic fluid deficits, which if present should be replaced with an isotonic solution.
Hypernatremia has been demonstrated to increase the minimum alveolar concentration for inhalation anesthetics in animal studies, but its clinical significance is more closely related to the associated fluid deficits. Hypovolemia accentuates any vasodilation or cardiac depression from anesthetic agents and predisposes to hypotension and hypoperfusion of tissues. Decreases in the volume of distribution for drugs necessitate dose reductions for most intravenous agents, whereas decreases in cardiac output enhance the uptake of inhalation anesthetics.
Elective surgery should be postponed in patients with significant hypernatremia (>150 mEq/L) until the cause is established and fluid deficits are corrected. Both water and isotonic fluid deficits should be corrected prior to elective surgery.
Hypoosmolality is nearly always associated with hyponatremia ([Na+] < 135 mEq/L). Table 49-5 lists rare instances in which hyponatremia does not necessarily reflect hypoosmolality (pseudohyponatremia). Routine measurement of plasma osmolality in hyponatremic patients rapidly excludes pseudohyponatremia.
Hyponatremia with a normal plasma osmolality
|
|
Hyponatremia invariably reflects water retention from either an absolute increase in TBW or a loss of sodium in relative excess to loss of water. The kidneys’ normal capacity to produce dilute urine with an osmolality as low as 40 mOsm/kg (specific gravity 1.001) allows them to excrete over 10 L of free water per day if necessary. Because of this tremendous reserve, hyponatremia is nearly always the result of a defect in urinary diluting capacity (urinary osmolality > 100 mOsm/kg or specific gravity > 1.003). Rare instances of hyponatremia without an abnormality in renal diluting capacity (urinary osmolality < 100 mOsm/kg) are generally attributed to primary polydipsia or reset osmoreceptors; the latter two conditions can be differentiated by water restriction.
Clinically, hyponatremia is best classified according to total body sodium content (Table 49-6). Hyponatremia associated with transurethral resection of the prostate is discussed in Chapter 31.
|
Normal total sodium content
|
Increased total sodium content
|
Progressive losses of both sodium and water eventually lead to extracellular volume depletion. As the intravascular volume deficit reaches 5-10%, nonosmotic ADH secretion is activated (see above). With further volume depletion, the stimuli for nonosmotic ADH release overcome any hyponatremia-induced suppression of ADH. Preservation of circulatory volume takes place at the expense of plasma osmolality.
Fluid losses resulting in hyponatremia may be renal or extrarenal in origin. Renal losses are most commonly related to thiazide diuretics and result in a urinary [Na+] greater than 20 mEq/L. Extrarenal losses are typically gastrointestinal and usually produce a urinary [Na+] of less than 10 mEq/L. A major exception to the latter is hyponatremia due to vomiting, which can result in a urinary [Na+] greater than 20 mEq/L. In those instances, bicarbonaturia from the associated metabolic alkalosis obligates concomitant excretion of Na+ with HCO3 to maintain electrical neutrality in the urine; urinary chloride concentration, however, is usually less than 10 mEq/L.
Edematous disorders are characterized by an increase in both total body sodium and TBW. When the increase in water exceeds that in sodium, hyponatremia occurs. Edematous disorders include congestive heart failure, cirrhosis, kidney failure, and nephrotic syndrome. Hyponatremia in these settings results from progressive impairment of renal free water excretion and generally parallels underlying disease severity. Pathophysiological mechanisms include nonosmotic ADH release and decreased delivery of fluid to the distal diluting segment in nephrons (see Chapter 29). The “effective” circulating blood volume is reduced.
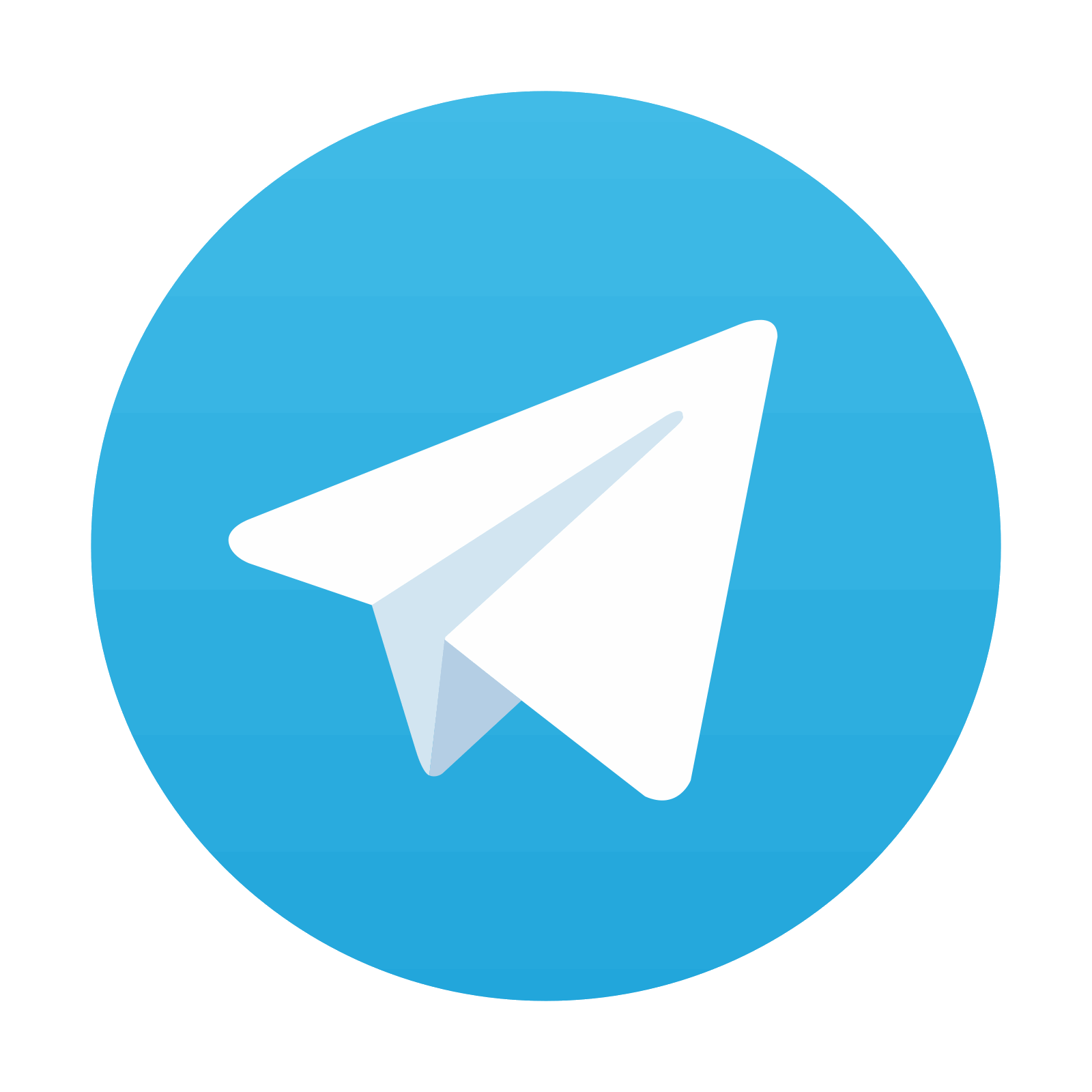
Stay updated, free articles. Join our Telegram channel
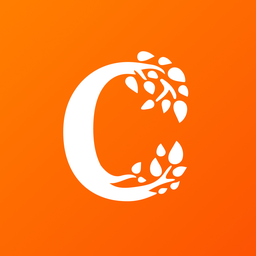
Full access? Get Clinical Tree
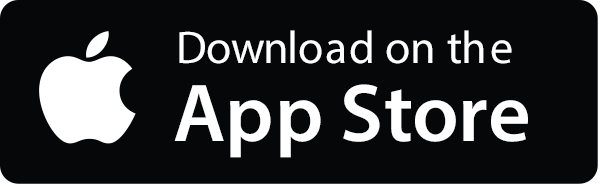
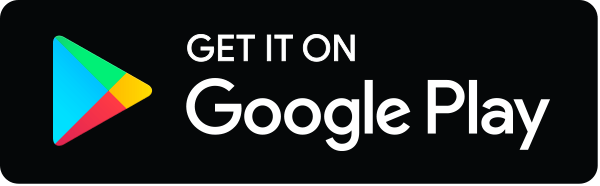