Data from National Institutes of Health: National Heart, Lung, and Blood Institute: NHLBI fact book, fiscal year 2012 (February 2013). Bethesda, MD, 2012, NHLBI.
These multiple issues in the aging trauma population have important implications for the reconsideration of resuscitation goals or endpoints, and specific, targeted management throughout their hospitalization.4 The following case study is used as a basis of discussion throughout this chapter.
Etiology of Traumatic Injuries
Injury without interruption of skin integrity is considered blunt trauma. Blunt trauma may be life threatening because the extent of the injury may be covert, making diagnosis difficult. Blunt forces transfer energy that causes tissue deformation. The nature of the injury is related to both the transfer of energy and the anatomic structures involved.8
Penetrating trauma refers to injury sustained by the transmission of energy to body tissues from a moving, projectile object that interrupts skin integrity, whereas blunt trauma produces tissue deformation by the transfer of energy. Penetrating trauma produces actual tissue penetration and may also cause surrounding tissue deformation based on the energy transferred by the penetrating object.8
Key Physiologic Concepts
Hemorrhagic Shock
Hemorrhagic shock is caused by a sudden loss of intravascular volume, as experienced in blood loss related to injury. Venous return to the heart is decreased, and this results in reduced cardiac output. Stimulation of the sympathetic nervous system and neurohormonal responses increases circulating blood volume to compensate for the blood loss (Figure 19-1). Until the hemorrhage is controlled and circulating volume is restored, the existing blood volume is shunted to the vital organs (heart, lungs, and brain), causing hypoperfusion to other organs such as the liver, stomach, and kidneys. Eventually, compensatory mechanisms become ineffective, causing cellular hypoperfusion and inability to meet cellular oxygen requirements for metabolism. The cells use anaerobic metabolism in an effort to meet their cellular adenosine triphosphate (ATP) requirements, resulting in lactic acidosis.
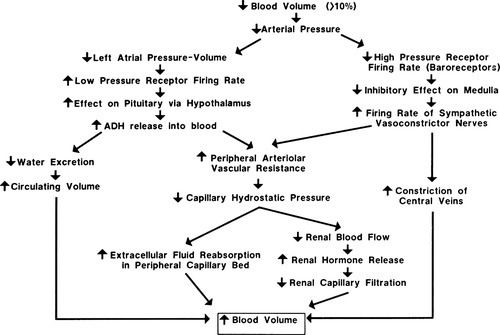
Sympathetic stimulation to increase heart rate, contractility, and systemic vascular resistance (SVR) escalates the workload of the heart. Ejection of a higher volume of blood against an increased afterload further stresses the myocardium, causing an increase in myocardial metabolism and myocardial oxygen consumption (MvO2). The continued lack of circulating volume reduces oxygen delivery to the heart, creating a vicious cycle. Circulatory collapse fails to provide end organ perfusion, with reduction in oxygen delivery, and forces a conversion to anaerobic (without oxygen) metabolism to meet cellular energy needs. Anaerobic metabolism cannot provide sufficient adenosine triphosphate (ATP) to meet energy demands; for further discussion on the impact of anaerobic metabolism see Chapter 6 and Figure 6-12. If the situation continues, myocardial fatigue, circulatory collapse, inadequate cell perfusion and ischemic damage may result in end organ failure (Figure 19-2).
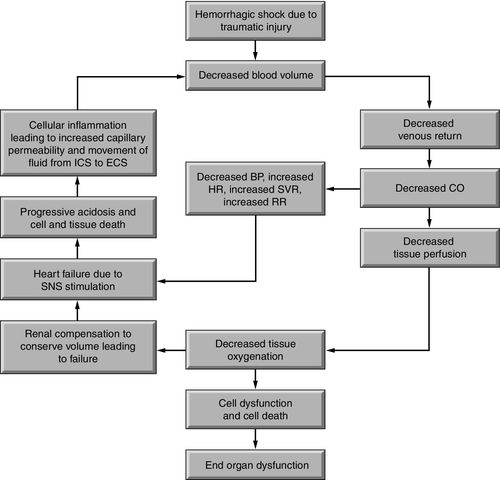
Systemic Inflammatory Response Syndrome
Once a shock state develops, the subsequent course may have more to do with the physiologic response to shock, including activation of the sympathetic nervous system, the inflammatory response, and the immune system, rather than with the initial cause of the shock. Thus, shock can be considered a derangement of compensatory mechanisms that results in further circulatory and respiratory dysfunction with subsequent multiple organ damage.
Activation of the inflammatory response causes the release of cytokines from macrophages such as tumor necrosis factor-alpha (TNF-α) and interleukin-1 (IL-1). Normally, tight junctions exist between the endothelial cells that line blood vessels. Proinflammatory cytokines disrupt these tight junctions, causing the endothelial cells to separate, increasing capillary permeability and plasma leak into the interstitial spaces. The coagulation system is activated because of the endothelial cell separation and exposure of the sub-basement endothelial membrane. Platelets aggregate and adhere to endothelial cells and sub-basement membrane, forming platelet plugs. Fibrin, the end product of the coagulation cascade, forms strands around the clot to give it stability and strength.
Proinflammatory cytokines also attract phagocytic white blood cells (WBCs) to the area and activate the complement cascade. The combination of WBC activity and complement proteins may result in elimination of the invading microorganism.9
Endothelial cells that line blood vessels are central to the development of a local inflammatory response. Endothelial cells provide an anticoagulant surface and control permeability of vessels.10 In a local inflammatory response, endothelial cells near the site of inflammation become activated as a result of mediators released by injured tissue cells. The activated endothelial cells express cell surface proteins that attract platelets and neutrophils. A procoagulant endothelial surface is formed in the area. Thus, in SIRS, increased coagulation, neutrophil aggregation, and impaired fibrinolytic mechanisms lead to microthrombi formation and reduced or obstructed capillary blood flow.11
WBCs, platelets, and activated endothelial cells release vasodilating substances such as nitric oxide (NO), histamine, and bradykinin. These substances promote additional capillary leak from blood vessels, which result in additional extravasation of plasma and coagulation factors.
In SIRS, the inflammatory response is systemic. The result is an overwhelming, unregulated inflammation with uncontrolled coagulation, disruption of capillaries, intravascular volume loss, maldistribution of circulating volume, and imbalance of oxygen supply and demand.9 Endothelial cells are activated in many vessels throughout the body, causing widespread extravasation of fluid into the interstitial compartment and systemic activation of the immune system and coagulation cascade (Figure 19-3).
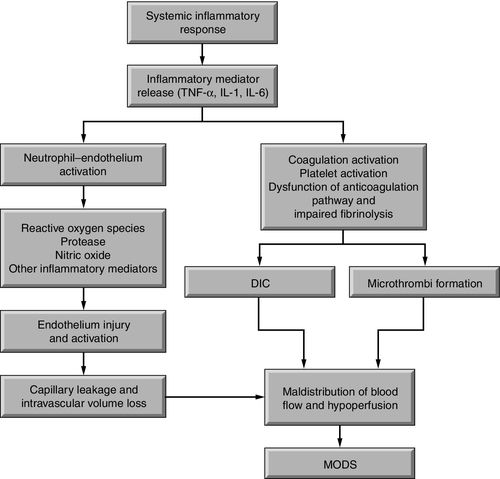
Substantial extravascular fluid accumulation and microthrombi formation in capillaries and in the interstitium decreases circulating blood volume, This cascade of events results in reduced perfusion of vital organs increasing the likelihood of multiple organ dysfunction syndrome (MODS) and death.
The complex interaction of SIRS mediators remains an active area of clinical research. Multiple mediators are believed to play a role in the maldistribution of blood flow, oxygen delivery and the consumption imbalance associated with SIRS and sepsis. Table 19-2 lists the key cellular mediators of SIRS and summarizes their activity.
Trauma Resuscitation Phase
Hemodynamic assessment in the trauma resuscitation area begins with a few basic indicators. Heart rate, noninvasive (cuff) blood pressure, and oxygen saturation measurements are taken upon patient arrival. Other hemodynamic parameters that can be measured in the trauma resuscitation area include central venous pressure (CVP), cardiac output and tissue oxygen saturation (StO2). Clinical laboratory tests are frequently obtained in the trauma resuscitation area. Common tests include a basic chemistry panel, complete blood cell count (CBC), coagulation profile, arterial blood gas (ABG) and lactate.
Hemodynamic Assessment Parameters
Heart rate is one of the simplest vital signs to measure in the trauma resuscitation area. Of the three hemodynamic parameters measured upon patient arrival in the trauma resuscitation area, heart rate is the most sensitive to blood loss and actual or potential hemodynamic instability. Because the sympathetic nervous system and neurohormonal responses are activated to increase circulating blood volume and compensate for the blood loss, as previously described (see Figure 19-1), even small volume losses can result in an increase in heart rate.8 Hemorrhage is not the only factor that can cause heart rate elevation in trauma patients. Pain is an expected complaint of any trauma patient and will cause an elevation in heart rate. Persistent tachycardia in the presence of adequate pain control should be interpreted as significant for volume loss until proven otherwise.
Blood pressure is not a reliable measure of actual end organ perfusion because of the multiple compensatory mechanisms activated by hypovolemia. In the resuscitation area noninvasive blood pressure via automated cuff blood pressure devices does help establish an important surrogate marker of perfusion until specific markers such as serum lactate, are obtained. Unlike heart rate, blood pressure abnormalities may not appear until significant blood loss has occurred.8 A patient who presents to the trauma resuscitation area with hypotension should be assumed to be in profound shock. Transient blood pressure changes in the trauma patient should be viewed as a marker of intravascular volume status, as opposed to end organ perfusion. The normotensive trauma patient who develops transient hypotension with the administration of analgesia or sedation is likely to be hypovolemic. Analgesics and sedatives blunt the sympathetic nervous system response to trauma and hypotension. If the patient has sustained significant blood loss, hypotension will result.
Direct arterial blood pressure measurement via an arterial catheter and pressure monitoring system is an option in the trauma resuscitation area, although not a practical early monitoring strategy. Arterial cannulation may be challenging in some patients who are in hypovolemic shock, as vasoconstriction, low blood pressure, and low intravascular volume all conspire to raise the difficulty of the procedure. While inserting the catheter, direct arterial blood pressure monitoring may be challenging. The advantage of arterial pressure monitoring is that it provides continuous and more accurate data regarding blood pressure than noninvasive automated blood pressure devices.12
Indirect automated cuff pressures overestimate blood pressure in hypotensive states. Arterial pressure monitoring may also provide information at lower blood pressure than noninvasive devices are able to measure. As will be discussed later, there are direct arterial pressure systems that can be utilized to monitor cardiac output.
Practitioners need to be cognizant of the trauma patient’s pre-existing medical conditions. For example, a patient with uncontrolled or untreated hypertension may experience the effects of hypotension at a significantly higher blood pressure than expected. Additionally, medications used to manage pre-existing conditions may significantly alter baseline hemodynamic parameters. A typical example is the patient taking a beta-blocker medication. A patient appropriately dosed on beta-blockers will not be able to elevate his or her heart rate as a compensatory response to blood loss.
Arterial Oxygenation Assessment
Arterial oxygen saturation is an important determinant of oxygen delivery. Assessment of arterial oxygen saturation by pulse oximetry (SpO2) provides additional information related to the patient’s hemodynamic status in the trauma resuscitation area. Oxygen saturation measurement reflects the amount of oxygen bound to hemoglobin that is available to the tissues and allows an estimation of the partial pressure of oxygen (PaO2) dissolved in the plasma. Blood loss does not shift the oxyhemoglobin dissociation curve, so on initial presentation, a saturation of 90% still correlates with a PaO2 of 60 mm Hg.13 Because oxygen saturation is not impacted by blood loss, a reading of 100% simply means that even in the face of severe hemorrhage, the available hemoglobin is fully saturated with oxygen. This should not be misinterpreted as adequate perfusion. In a profound shock state, the body can deliver fully saturated hemoglobin to the tissues, but it may be insufficient to meet metabolic requirements or the cells may not extract the oxygen. New technology that helps measure the oxygen levels of peripheral tissue has been developed and will be discussed later in this chapter.
SpO2 may also be misleading in other conditions. Oxygen saturation via SpO2 may be difficult to assess in the patient with significant vasoconstriction, as most monitors are designed to measure the saturation in peripheral digits such as fingers. As arteries constrict, blood flow to the digits is reduced, and the sensor may not be able to detect an adequate signal. Hypothermia causes similar difficulties with accurate measurement. Modern pulse oximeters include both waveform and signal quality indicators; oxygen saturation is most accurate in the presence of an appropriate waveform and high signal quality index. Another condition that impacts SpO2, measurement in trauma patients, particularly if involved in a fire, is carbon monoxide inhalation and the formation of dyshemoglobins. In carbon monoxide poisoning cases, hemoglobin preferentially binds to carbon monoxide rather than to oxygen. As all of the hemoglobin’s binding sites are filled, the oxygen saturation sensor will report a saturation level near 100%, even though the hemoglobin is bound with a compound that cannot contribute to tissue oxygen metabolism. Refer to Figure 6-7 and Table 6-2 in Chapter 6 for more information about measurement of oxygen saturation and carbon monoxide. ABG measurement via co-oximetry in the laboratory will provide accurate information about oxygen availability in these patients.13
Tissue Oxygen Assessment
Tissue oxygen saturation (StO2) is a relatively new parameter for use in trauma patients. Similar to SpO2, this technology uses near-infrared spectroscopy to measure the oxygen saturation via a noninvasive, single-use sensor placed on the thenar eminence (thumb muscle). Unlike SpO2, which evaluates the percent hemoglobin saturated with oxygen in the arterial circulation, StO2 evaluates the hemoglobin saturation of blood cells in the capillary beds of underlying tissues where cellular gas exchange occurs.
This parameter provides an assessment of perfusion as it evaluates oxygen uptake at the tissue level rather than oxygen delivery. A normal value for StO2 is in the range of 86% to 90%; the lower the value, the more severe is the hypoperfusion of the tissue bed being monitored. Values in the range of 40% to 55% suggest severe shock in trauma patients.14 Tissue oxygen saturation is well correlated with other markers of perfusion such as lactate and base deficit.15 Recent studies have shown a correlation between StO2 and mortality in patients requiring a massive transfusion.16 Because it is noninvasive, StO2 may be useful in the prehospital setting by paramedics or military medics. A recent study that examined the feasibility of prehospital use of StO2, reported that baseline StO2 measurements did not differ between survivors and nonsurvivors. However, when a vascular occlusion test was incorporated, a comparison of the pre- and postocclusion StO2 was predictive of in-hospital mortality.17
Central Venous Pressure Assessment
Less commonly measured hemodynamic parameters during the resuscitation include CVP and cardiac output. CVP can provide some information about intravascular volume status and preload; however, it is not a reliable source of data to predict the patients need for additional volume as described in the Critical Care Phase section of this chapter. In addition, the upper torso is not a preferred site for central venous access during resuscitation. The relative complexity of vascular venous access makes the subclavian approach less than ideal and many patients present with the potential for cervical injury, thus eliminating the internal jugular as a site for cannulation.
Cardiac Output Assessment
Cardiac output monitoring in trauma patients is more often implemented in the critical care unit. Use of arterial pressure waveform-based, less-invasive cardiac output monitoring (described in the Critical Care Phase) has not yet been widely adopted in the trauma resuscitation area. However, in trauma patients with pre-existing cardiovascular disease, monitoring cardiac output and stroke volume variation via an arterial catheter may be useful to avoid complications of overly aggressive volume administration. See Chapter 12 for more information on arterial waveform–based cardiac output monitoring.
Laboratory Assessments
Evaluation of shock in the trauma patient requires assessment of multiple laboratory tests in conjunction with hemodynamic monitoring. A typical battery of laboratory tests in the trauma resuscitation area includes basic chemistries, a CBC, and a coagulation panel consisting of partial thromboplastin time (PTT), prothrombin time (PT), and international normalized ratio (INR). These blood tests provide valuable information about a patient’s baseline status but should not be utilized as the sole guideline for management in a severely injured trauma patient. The decision to transfuse is based on clinical presentation, heart rate, and blood pressure, as well as hemoglobin and hematocrit. Similarly, the decision to use fresh frozen plasma and platelets in the severely injured trauma patient is not determined by the results of the INR or the platelet count. An exception may be the patient with a severe traumatic brain injury. Brain tissue damage could activate the coagulation cascade, which may lead to clotting factor consumption and coagulopathies. Brain injured patients may require directed interventions with recombinant factor VII, fresh frozen plasma, and platelets to prevent or reduce further intracranial bleeding.11
Initial laboratory studies provide a measure of the adequacy of cellular oxygenation through evaluation of serum lactate or base deficit. These laboratory tests provide an early indication of end organ perfusion. Hypoperfusion of tissues leads to cellular hypoxia that results in anaerobic metabolism (which produces 2 ATP molecules versus 36 in aerobic metabolism), and pyruvate. Pyruvate is converted into lactic acid. Large quantities of hydrogen ions are generated in this process causing serum pH to decrease. As oxygen availability decreases to below metabolic requirements with hemorrhage, lactic production increases, and serum lactate measurements rise above the normal 2.2 millimeters per liter (mm/L). Elevated serum lactate levels in a severely hypovolemic patient may indicate the severity of shock, which is especially useful in patients who may show little hemodynamic evidence of their shock state, for example, those in compensated shock or on beta-blocker therapy.
Serum lactate levels in patients in shock have been demonstrated to correlate with outcome and have been utilized to guide resuscitation. A recent study found that in a wide variety of traumatized patients, both initial lactate and lactate clearance provide important prognostic information over and above traditional clinical predictors of mortality.18 As reported, one of every eight patients who are not hypotensive but have a lactate of 4.0 milligrams per deciliter (mg/dL) or greater die, as do patients who have poor lactate clearance. A significant decrease in mortality is seen among patients whose lactate returns to normal levels within 24 hours of injury compared with those whose serum lactate level requires longer than 24 hours to normalize.19
Other physiologic conditions associated with traumatic injuries in addition to shock may cause an elevation in lactic acid levels. For example, alcohol toxicity or traumatic brain injury could cause seizures and result in an increased lactate level.20,21 Thus, evaluation of the serum lactate level of a seriously injured patient who presents with post-injury seizures may be challenging.
Base deficit is another useful marker of end organ perfusion in the severely injured patient. Base deficit represents the actual deficit of base in the bloodstream in a patient with compensated or uncompensated acidosis. The production of lactic acid by tissues in anaerobic metabolism causes metabolic acidosis, and thus a base deficit, to develop. Even patients with compensated metabolic acidosis may have a measurable base deficit. Because base deficit is a component of ABG analysis, practitioners will also gain valuable information from the other components of blood gas analysis, including pH and PaO2.13 See Chapter 6 for additional information about arterial oxygen and acid–base monitoring.
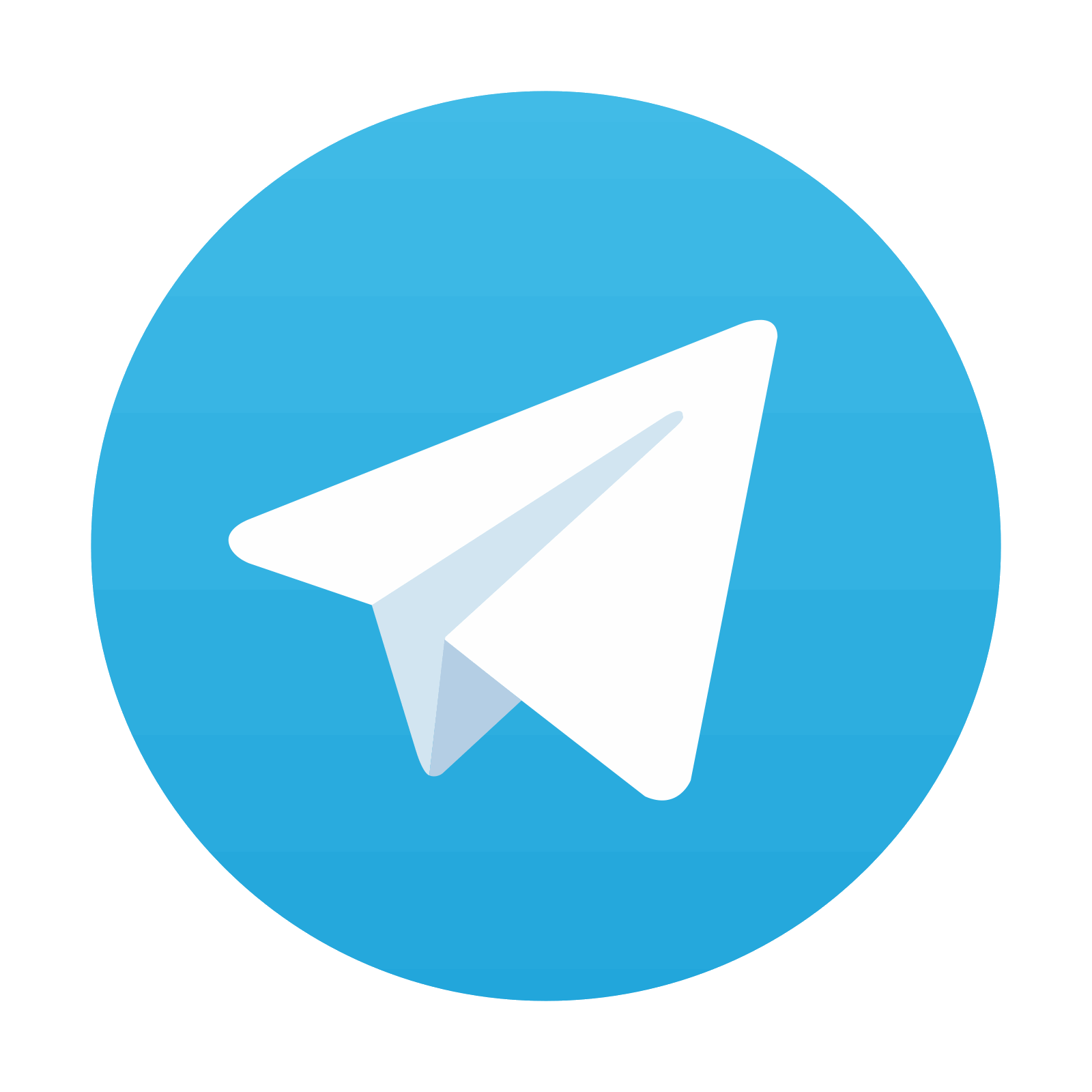
Stay updated, free articles. Join our Telegram channel
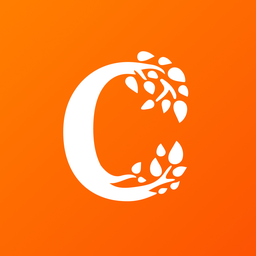
Full access? Get Clinical Tree
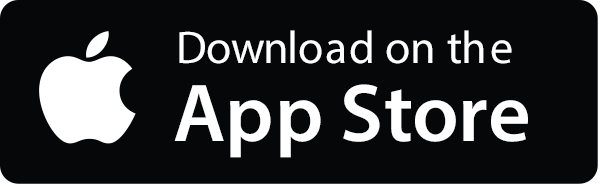
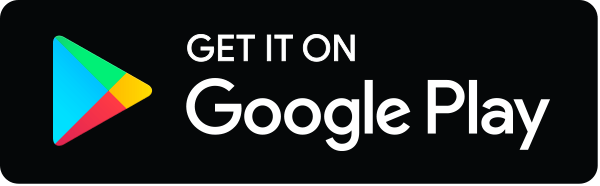