Conventional Positive-Pressure Breath-Delivery Strategies and Modes
The first generation of positive-pressure mechanical ventilators were simple high-pressure gas regulators on which clinicians could set the circuit pressure and the breathing frequency. In the middle of the twentieth century, more sophisticated devices appeared that allowed direct clinician control of flow and volume along with breath timing and expiratory pressure. As ventilator design improved and microprocessors became available, feedback mechanisms appeared that could provide automatic adjustments in these set variables depending upon a variety of conditions.1 A simple example was the use of a patient-effort sensor to adjust the number of mechanical breaths provided during assist-control modes or synchronized intermittent mandatory ventilation.2–4 A variation on this breath rate feedback mechanism was mandatory (or minimum) minute ventilation, which used minute ventilation to adjust the number of positive-pressure breaths delivered.5 At the same time, the development of flow control valves that could be adjusted based on a clinician-selected airway-pressure target appeared.6–9 This gave clinicians the choice of using either set flow-volume-targeted modes (volume assist-control ventilation; volume-targeted synchronized intermittent mandatory ventilation [volume SIMV]) or pressure-targeted modes (pressure-targeted assist-control ventilation [PACV]; pressure support; pressure SIMV). Taken together, these flow-targeted, volume-targeted, and pressure-targeted strategies comprise what is commonly referred to today as “conventional” mechanical ventilation.
In the late twentieth and early twenty-first century, increasingly complex and clever feedback mechanisms for these conventional breaths have been introduced. The behavior of these features is made more understandable if one considers that all positive-pressure breaths can be described by three variables: the trigger variable (what initiates a breath—usually an effort sensor or a machine timer); the target variable (what governs gas delivery—usually either a set flow or a set pressure target); and the cycle variable (what terminates the breath—usually a set time, flow, or volume).6,8 These newer feedback systems are designed to adjust one or more of these breath-delivery variables based on prescribed algorithms to provide more physiologically targeted and patient interactive ventilatory support. In addition, there has also been the development of feedback mechanisms to adjust the fractional inspired oxygen concentration (FIO2) and positive end-expiratory pressure (PEEP). These feedback mechanisms are often considered “partial” closed-loop conventional mechanical ventilation. The clinician, however, cannot expect these features to provide safe and effective support automatically. Rather, the clinician must understand their rationale, design principles, lung-protective targets, and evidence-based outcomes to apply them properly. These are the focus of the remainder of this chapter.
Feedback Control of Combination Pressure-Targeted and Flow-Targeted Breaths
The advantage of a flow-targeted, volume-cycled breath is that a guaranteed volume is delivered with every breath (even though applied airway pressures may change). The advantages of a pressure-targeted breath (either time- or flow-cycled) are that an airway pressure limit is guaranteed (even though volumes may change), that the rapid initial flow may enhance gas mixing, and that the variable-flow pattern may enhance patient–ventilator synchrony.7,10,11
Over the last two decades, a number of engineering innovations have attempted to combine these features by producing feedback algorithms that allow some control of volume with pressure targeting and some control of pressure with flow targeting. Collectively, these are often referred to as hybrid breaths or dual-control breaths.12–15 On the current generation of mechanical ventilators, there are two basic approaches to providing these types of breaths: dual control within a breath (DCWB; intrabreath control) and dual control from breath to breath (DCBB; interbreath control). The former uses a measured flow input to switch from pressure targeting to flow targeting in the middle of the breath. The latter uses a measured volume input to manipulate the pressure level of subsequent pressure-targeted breaths.
The currently available DCWB breath begins with either patient or machine triggering and is followed by a pressure-targeted flow-delivery algorithm.16 There is thus a high initial flow to rapidly pressurize the airway and then subsequent flow adjustments according to respiratory system mechanics and patient effort to maintain the target pressure. As the lungs fill, flow decelerates until a flow-cycling mechanism terminates the breath. In these respects, this breath type is similar to the pressure support breath. Unlike pressure support, however, the clinician also must set a minimum tidal volume, flow, and backup rate with the DCWB breath. These backup settings take over control of the breath should the pressure-targeted flow drop below the minimum required to deliver the set tidal volume in the allotted inspiratory time. The breath thus begins like pressure support and can either flow cycle like pressure support (if the volume meets or exceeds the set minimum) or volume cycle like a flow-targeted breath (if necessary to deliver the set volume).12–14,16
The reasoning behind DCWB breaths is that the high initial flow provides better gas mixing and also reduces flow dyssynchrony during assisted breaths, whereas the volume guarantee ensures a constant tidal volume (VT).10,17–21 DCWB breaths thus can be considered to be “more comfortable” flow-targeted, volume-cycled breaths. Alternatively, they could be considered pressure support with a VT “safety net.”16
DCWB breath algorithms exist on several ventilators, but their clinical role remains unclear because the few studies looking at this strategy have generally focused only on device performance.16,22 Indeed, DCWB mode use is driven primarily by a clinician’s belief in the underlying concept. In recent years, DCWB modes have gradually disappeared and have been replaced with the simpler to use DCBB approach described below.
DCBB techniques use standard pressure-targeted breaths (either pressure support or PACV), but the ventilator has the ability to adjust the pressure target according to a clinician-set VT and the delivered VT of previous breaths.13,14,23–26 When DCBB breaths are exclusively supplied with time cycling, the mode is commonly referred to as pressure-regulated volume control, although there are a number of proprietary names (e.g., Dräger’s Autoflow, Covidien’s VC+, Hamilton’s Adaptive Pressure Ventilation). When DCBB breaths are supplied exclusively with flow cycling, the mode is commonly referred to as volume support. Some ventilators will switch between these two breath types depending on the number of patient efforts (e.g., Maquet’s Automode). With all of these modes, instead of setting a target pressure, the clinician selects a VT. The ventilator then delivers one or more “test breaths” with a small amount of inspiratory pressure. The VT exiting the ventilator is measured, and total respiratory system compliance is calculated. Thereafter, each subsequent breath uses the previous calculation of system compliance to manipulate the ensuing pressure target to achieve the desired VT (Fig. 15-1). The maximum pressure change from breath to breath on most systems generally is limited to a few centimeters of water (<3 cm H2O) to prevent large swings in pressure and volume. The volume signal used for DCBB feedback control is not exhaled VT but VT exiting the ventilator. This prevents a runaway effect, which could occur if a leak in the circuit prevented accurate measurement of exhaled VT.
Figure 15-1
Behavior of DCBB breaths in a lung model simulation of changing lung compliance. In both the top and bottom panels, pressure (Paw), flow, and volume (Vol) are plotted over time. The target tidal volume in both panels is 600 mL. In the top panel, lung compliance decreases after the fourth breath. Initially, there is a drop in tidal volume, but then the DCBB algorithm gradually increases the target inspiratory pressure to restore the volume. In the bottom panel, lung compliance increases after the fourth breath. Initially, there is an increase in tidal volume, but then the DCBB algorithm gradually reduces the inspiratory pressure target to restore the volume. (Reproduced, with permission, from Branson et al: The role of ventilator graphics when setting dual-control modes, Respir Care Feb;50(2); 187-201; 2005.)
In volume support, the flow-cycling criterion is either manufacturer-specific (e.g., 25% to 35% of peak flow) or, on many newer machines, clinician-adjustable. A secondary cycling mechanism may be present on some devices if inspiratory time exceeds a certain fraction (e.g., 80%) of a set total cycle time. Also, as with other pressure-targeted breaths, a rate-of-rise adjustment usually is available on DCBB breaths. When time-cycled DCBB breaths are interspersed with either spontaneous unsupported or pressure-supported breaths, or when flow-cycled DCBB breaths are interspersed with conventional PACV breaths, algorithms similar to SIMV or mandatory minute volume are often used to determine which breath type will be delivered.27
Both animal and human studies show that DCBB breaths function as designed using both flow-cycled and time-cycled approaches.23–29 These studies emphasize that the DCBB breath is similar to other pressure-targeted breaths with enhanced gas mixing and better patient–ventilator flow synchrony as compared with flow-targeted, volume-cycled breaths. They also confirm that DCBB breaths do provide a volume guarantee without untoward side effects. Not surprisingly, several of these studies showed lower peak pressures with DCBB breaths than with volume assist-control breaths.23,24 This, however, is a finding consistent with the decelerating flow patterns of all pressure-targeted breaths (e.g., pressure support and PACV).
The role of DCBB modes in providing “lung-protective strategies” for patients with acute lung injury is unclear. Lung-protective strategies are based on data from numerous animal studies and clinical trials that low tidal volumes and low end-inspiratory lung-stretching pressures reduce ventilator-induced lung injury.30 Setting a low tidal volume (e.g., 6 mL/kg ideal body weight) directly with flow-targeted volume-cycled breaths is the most reliable way to assure that a desired low tidal volume is delivered. Some researchers argue, however, that the set flow breath during low tidal ventilation may be particularly uncomfortable and that the variable flow feature of pressure-targeted breaths may synchronize better with patient efforts under these circumstances.31,32 Assuring a guaranteed tidal volume with a pressure-targeted DCBB breath would seem an attractive way to combine these features. Kallet et al addressed this issue. Although they found that DCBB breaths did provide a more reliable small tidal volume ventilatory pattern than pure pressure assist control, in a minority of patients up to 14% of tidal volumes were above the desired target value.33,34 Whether this variability is an acceptable trade-off for improved comfort during lung-protective ventilation needs further study.
DCBB breaths have also been evaluated during the ventilator-withdrawal process. Several evidence-based consensus groups have found that routine use of daily spontaneous breathing trials is critical in assessing ventilator discontinuation potential.35 Less clear, however, is the role of gradually reducing ventilator support (“weaning”), either before or between spontaneous breathing trials. If weaning is desired, however, the DCBB modes have some conceptual appeal. Theoretically, the DCBB breath could be used to automatically reduce applied inspiratory pressure as the patient’s ability to breathe improved. Conversely, inspiratory pressure would increase if patient effort diminished or respiratory system mechanics worsened. Flow-cycled DCBB breaths, either alone or in combination with time-cycled DCBB breaths or various SIMV and/or pressure-support modes, have been used in several weaning studies. In general, they have performed as well as (or sometimes better than) standalone SIMV or pressure-support protocols, especially in the rapidly recovering (e.g., postoperative) patient.29,36–39 A common finding in these weaning studies is that the DCBB breath modes required fewer ventilator manipulations. One must be cautious, however, in interpreting these weaning studies. A number of clinical trials show that the SIMV or SIMV + pressure-support control strategies delay weaning inappropriately as compared with spontaneous breathing trials or stand-alone pressure-support strategies.40,41 A more appropriate evaluation of DCBB breath-weaning strategies would be a comparison with spontaneous breathing trials delivered according to a protocol.35,42,43
Unfortunately, the simplicity of the DCBB modes for weaning may produce problems.44 For instance, if the clinician-set volume is excessive for patient demand, a recovering patient may not attempt to take over the work of breathing for that volume and thus support reduction and weaning may not progress. In addition, if the pressure level increases in an attempt to maintain an inappropriately high set tidal volume in the patient with airflow obstruction, intrinsic PEEP may result. On the other hand, a patient may receive inadequate support if the clinician-set tidal volume is inadequate for patient demand. Under these conditions, a patient will perform excessive work to maintain a patient-desired tidal volume while the inspiratory pressure is being reduced because volume exceeds the clinician setting. Clinicians need to be aware of the behavior of DCBB breaths under a variety of circumstances to properly use this mode.
Airway occlusion pressure (P0.1),45 oxygen saturation (SpO2),37–39 and end-tidal CO2 concentrations46,47 have been incorporated into DCBB mode-control algorithms to adjust either the target VT or the breath-delivery pattern. The one system that is commercially available uses end-tidal CO2 and respiratory rate along with the tidal volume to adjust the applied inspiratory pressure.47 Known by the proprietary trade name SmartCare (Dräger), the computerized feedback system attempts to find an inspiratory pressure support that maintains the respiratory rate and tidal volume in a clinician-set “comfort zone.” The end-tidal CO2 serves as a backup signal to assure adequate ventilation. The system is designed to wean the inspiratory pressure to as low a level as possible within these boundaries and then alert the clinician to perform a spontaneous breathing trial when this pressure reaches 9 cm H2O.
A number of small observational trials have been done showing that the system did, indeed, keep patients in the clinician-selected “comfort zone” for 95% of the time.46,47 In a larger randomized clinical trial, this approach appeared to remove ventilator support quicker than “physician-controlled” weaning.48 Unfortunately, this control group did not have a protocolized spontaneous breathing trial approach, and thus may have had support removal delayed. Indeed, a subsequent trial noted that in the presence of an active protocol for discontinuing mechanical ventilation, automated withdrawal offered no advantage.49 Even if it is not superior, however, an automated system that is “just as good” as clinicians could have applications in settings with rapidly recovering patients or low availability of clinicians to make frequent assessments.
Feedback Control of Ventilator Breath Delivery Based on Respiratory System Mechanics
A novel approach to automated feedback control of ventilator support combines the DCBB principle with an integrated VT, frequency and inspiratory-to-expiratory (I:E) ratio algorithm based on respiratory system mechanics. Known as adaptive lung ventilation or adaptive-support ventilation (ASV, Hamilton Medical),50–55 the breath-control algorithm attempts to partition the frequency, tidal volume, and I:E ratio so as to minimize ventilator–patient inspiratory work and intrinsic PEEP. ASV does this by calculating respiratory system mechanics using several “test breaths.” It then uses a “minimal work” calculation to set the frequency–tidal volume pattern that minimizes the combined resistance and compliance components of work. The clinician must input the patient’s predicted body weight and the percent of desired minute ventilation. The predicted body weight frames the tidal volume range, avoiding volutrauma and hypoventilation. The predicted needed minute volume is 0.1 L/kg/min. As an example, a 70-kg patient set at 100% would receive a 7 L minute volume. If the percent minute volume was set to 125%, in the presence of increased physiologic dead space the minute ventilation would be 8.75 L. The ASV algorithm then uses a measurement of the expiratory time constants (RCe = resistance × compliance) to ensure an inspiratory time of at least one RCe and an expiratory time of at least three RCes. The actual formula is:
where RC is the respiratory time constant (the product of resistance R and compliance C), and VD are alveolar ventilation and dead space ventilation, respectively, and a is a constant that depends on the flow waveform.
Boundary rules exist to prevent excessive (runaway) settings (Table 15-1). Clinicians must set the desired minute ventilation and the proportion of that minute ventilation that the machine is to supply. Ideal body weight also can be used to calculate the desired minute ventilation based on metabolic demands and predicted dead space. Clinicians also must set the PEEP and FIO2.
Parameter | Minimum | Maximum |
---|---|---|
Inspiratory pressure (cm H2O) | 5 above baseline airway pressure (PEEP/CPAP) | 10 below Pmax alarm setting |
Tidal volume (mL) | 4.4 · IBW | 15.4 · IBW or ![]() |
Target respiratory rate (bpm) | 5 bpm | 22 bpm · % min vol/100 (if IBW > 15 kg) |
45 bpm · % min vol/100 (if IBW < 15 kg) | ||
Mandatory breath rate (bpm) | 5 bpm | 60 bpm |
Inspiratory time (s) | 0.5 s or 1 · RCe, whichever is longer | 2 s |
Expiratory time (s) | 2 · RCe (possibly 3 · RCe) | 12 s |
I:E ratio range | 1:4 | 1:1 |
ASV as a pure control mode has been evaluated in a number of ways. Initial lung-model testing56 demonstrated that the ASV algorithm responded properly to abrupt changes in lung mechanics. Several early clinical studies have compared initial ASV settings with traditional clinician-selected settings and have found that ASV tends to select a lower tidal volume and faster rate (and thus lower inspiratory pressures) than do clinicians.56–59 Two other early studies suggest that ASV also appropriately adapts to changes in patient position and the change from double- to single-lung ventilation during anesthesia.55–61 One other study suggested that the I:E algorithm of ASV produced less air trapping in patients with chronic obstructive pulmonary disease.62 Longer-duration clinical studies with ASV show that the algorithm provides adequate ventilator support in anesthetized patients,56,59 as well as in patients with respiratory failure.63
More recent evaluations of ASV, however, have focused on its ability to provide appropriate lung-protective small tidal volumes. Indeed, when respiratory system compliance is poor, the ASV algorithm supplies a protective low tidal volume ventilator pattern similar to that recommended by the ARDS Network.54 Problems arise, however, when respiratory system compliance is less deranged (e.g., patients with milder forms of acute lung injury). Under these conditions, the ASV algorithm tends to deliver tidal volumes often in excess of 10 mL/kg ideal body weight.45,64 The clinical significance of this is unknown but the potential harm from this should be considered by clinicians wishing to use this mode.
ASV also might be considered an automatic weaning mode because the algorithm responds with lower pressures and fewer mandatory breaths as patient effort increases. When spontaneous efforts occur with ASV, the algorithm continues to try to conform to the minimal work tidal volume considerations above and in that sense resembles the feedback features of volume support noted above.65
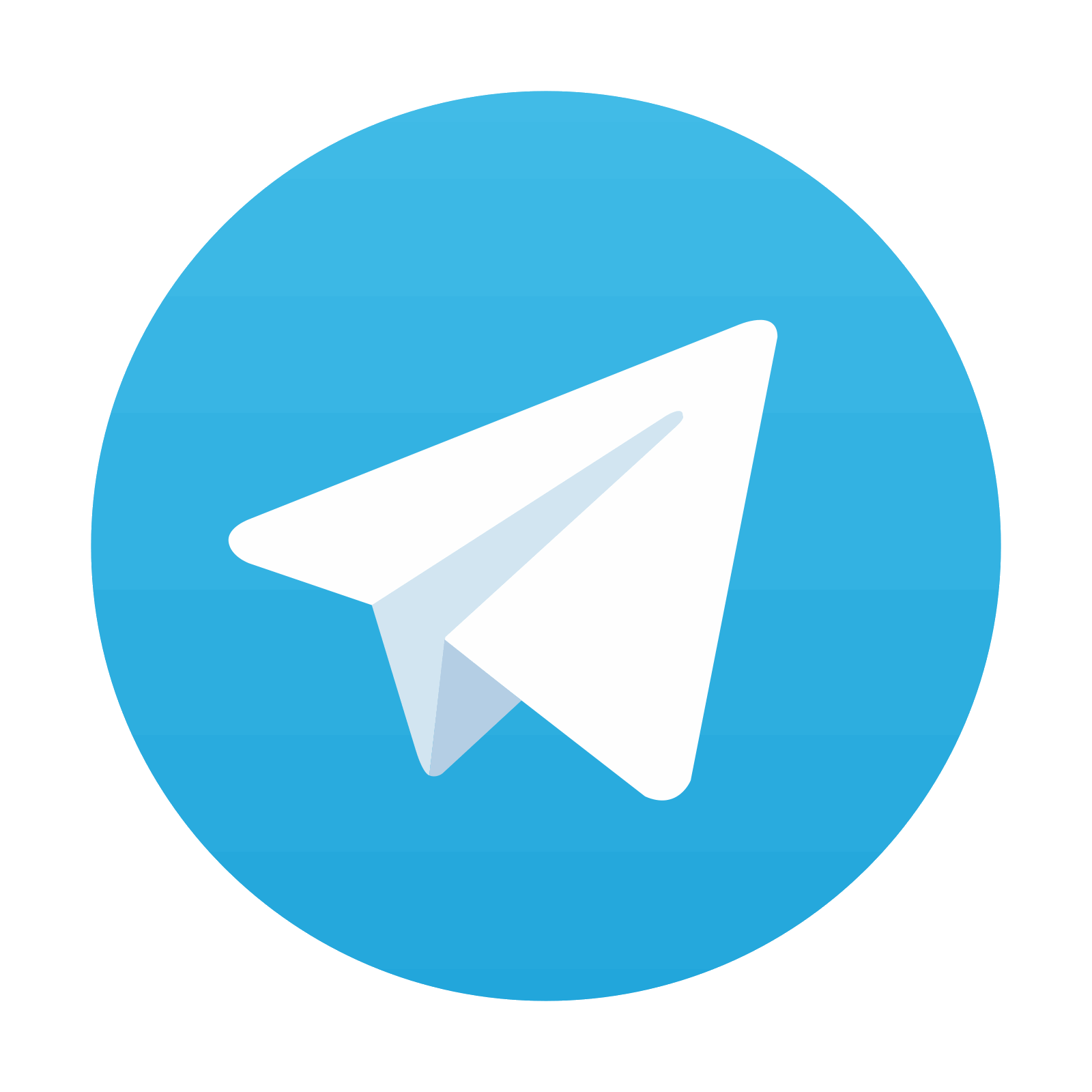
Stay updated, free articles. Join our Telegram channel
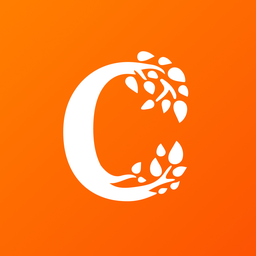
Full access? Get Clinical Tree
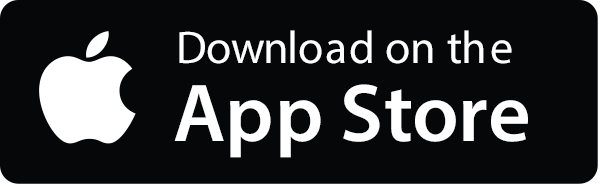
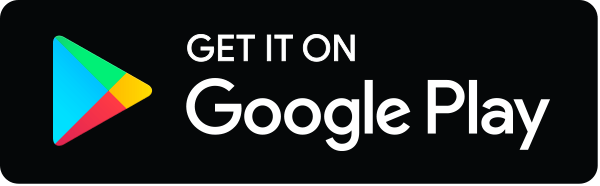
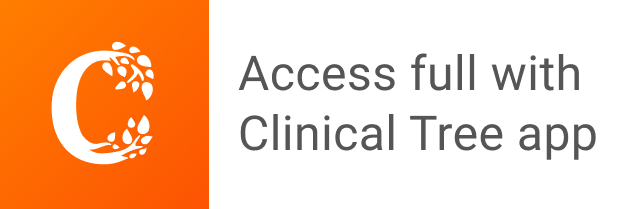