Key Concepts
Brain death criteria can be applied only in the absence of hypothermia, hypotension, metabolic or endocrine abnormalities, neuromuscular blockers, or drugs known to depress brain function.
Hyperoxia and hypoxia are risk factors, but not the primary causes of retinopathy of prematurity (ROP). Neonates’ risk of ROP increases with low birth weight and complexity of comorbidities (eg, sepsis).
Pressure control ventilation (PCV) is similar to pressure support ventilation in that peak airway pressure is controlled but is different in that a mandatory rate and inspiratory time are selected. As with pressure support, gas flow ceases when the pressure level is reached; however, the ventilator does not cycle to expiration until the preset inspiration time has elapsed.
The disadvantage of conventional PCV is that tidal volume (Vt) is not guaranteed (although there are modes in which the consistent delivered pressure of PCV can be combined with a predefined volume delivery).
When compared with orotracheal intubation, nasotracheal intubation may be more comfortable for the patient and more secure (fewer instances of accidental extubation).
When left in place for more than 2-3 weeks, both orotracheal and nasotracheal tubes predispose patients to subglottic stenosis. If longer periods of mechanical ventilation are necessary, the tracheal tube should generally be replaced by a cuffed tracheostomy tube.
The major effect of positive end-expiratory pressure (PEEP) on the lungs is to increase functional residual capacity (FRC). In patients with decreased lung volume, appropriate levels of either PEEP or continuous positive airway pressure (CPAP) will increase FRC and tidal ventilation above closing capacity, will improve lung compliance, and will correct ventilation/perfusion abnormalities.
A higher incidence of pulmonary barotrauma is observed with excessive PEEP or CPAP, particularly at levels greater than 20 cm H2O.
Maneuvers that produce sustained maximum lung inflation such as the use of an incentive spirometer can be helpful in inducing cough as well as preventing atelectasis and preserving normal lung volume.
While injury from high inspired oxygen concentrations has not been conclusively demonstrated in humans, Vt of 12 mL/kg was associated with greater mortality than Vt of 6 mL/kg and plateau pressure of less than 30 cm H2O in patients with acute respiratory distress syndrome.
Early elective tracheal intubation is advisable when there are obvious signs of heat injury to the airway.
The criteria developed by the Acute Kidney Injury Network are now most often used to stage acute kidney injury (AKI). AKI is diagnosed by documenting an increase in serum creatinine of more than 50%, or an absolute increase of 0.3 mg/dL, and a reduction in urine output to less than 0.5 mL/kg/h for 6 h or longer, with all findings developing over 48 h or less.
Age greater than 70 years, corticosteroid therapy, chemotherapy of malignancy, prolonged use of invasive devices, respiratory failure, kidney failure, head trauma, and burns are established risk factors for nosocomial infections.
Systemic venodilation and transudation of fluid into tissues result in a relative hypovolemia in patients with sepsis.
Critical Care: Introduction
Critical care medicine deals with potentially life-threatening illnesses. Anesthesiologists played a major role in developing this multidisciplinary subspecialty. Relative to most other physicians, anesthesiologists have greater expertise in airway management, mechanical ventilation, drug and fluid resuscitation, and advanced monitoring techniques that are central to effective care in critical illness. Moreover, the emphasis in anesthesia on physiology, pathophysiology, and pharmacology, as well as on rapid diagnosis and treatment of acute physiological derangements, provides an excellent foundation for a career in evaluating and treating patients with critical illness. The critical care physician (or “intensivist”) also requires broad knowledge that crosses internal medicine, surgery, pediatrics, neurology, emergency medicine, and palliative care. Unlike most subspecialty education, which tends to emphasize a single organ system, intensive care fellowships provide experience in treating patients with systemic inflammatory response syndrome (SIRS) and the related multiple organ dysfunction syndrome (MODS). The American Boards of Anesthesiology, Internal Medicine, Pediatrics, and Surgery, recognizing these requirements, sponsor specialized training for certification in critical care medicine. Clinicians who have such certification are increasingly recognized by multinational corporations and organizations as making important contributions to the outcomes of hospitalized patients.
This chapter provides an abbreviated survey of critical care medicine. Many topics relevant to critical care are covered in other chapters; only important topics not presented elsewhere will be presented.
AC | Assist-control (ventilation) |
AKI | Acute kidney injury |
AMI | Acute myocardial infarction |
APACHE | Acute Physiology and Chronic Health Evaluation |
APRV | Airway pressure release ventilation |
ARDS | Acute respiratory distress syndrome |
CMV | Continuous mandatory ventilation |
CPAP | Continuous positive airway pressure |
CRRT | Continuous renal replacement therapy |
EGD | Esophagogastroduodenoscopy |
FENa+ | Fractional excretion of filtered sodium |
FIO2 | Fraction of inspired oxygen |
FRC | Functional residual capacity |
HFJV | High-frequency jet ventilation |
HFV | High-frequency ventilation |
I:E | Inspiratory:expiratory (ratio) |
ILV | Independent lung ventilation |
IMV | Intermittent mandatory ventilation |
IPAP | Inspiratory positive airway pressure |
MMV | Mandatory minute ventilation |
MODS | Multiple organ dysfunction syndrome |
Pplt | Plateau pressure |
PCV | Pressure control ventilation |
PEEP | Positive end-expiratory pressure |
PSV | Pressure support ventilation |
ROP | Retinopathy of prematurity |
RSBI | Rapid shallow breathing index |
SIMV | Synchronized intermittent mandatory ventilation |
SIRS | Systemic inflammatory response syndrome |
TISS | Therapeutic Intervention Scoring System |
Vd | Volume of distribution |
Vt | Tidal volume |
Economic, Ethical, & Legal Issues in Critical Care
High-quality critical care is very expensive; poor-quality critical care is even more expensive. Intensive care unit (ICU) beds constitute only 10% of all beds in most hospitals yet account for a large fraction of hospital expenditures. If this cost is justified, clear reductions in morbidity or mortality should be readily demonstrable. Unfortunately, confirmatory studies are few and typically flawed by the use of historical controls. A method of reliably identifying those patients who will benefit most from intensive care is needed. Several scoring systems based on the severity of physiological derangements and preexisting health have been used, such as the Acute Physiology and Chronic Health Evaluation (APACHE) and Therapeutic Intervention Scoring System (TISS), but while all reliably identify “sicker” patients none reliably identifies the very sick but recoverable patients for whom intensive care is intended. Survival is generally inversely related to the severity of illness and number of organ systems affected. The Society of Critical Care Medicine has established Project Impact, a system that allows ICUs to compare their outcomes and the care they provide against a national and international network of ICUs.
The high cost of critical care medicine has led to economic constraints being applied by governments and third-party payers. At the same time an increased awareness of ethical and legal issues has changed the practice of critical care medicine.
Decisions about when to initiate or terminate treatment can be difficult. Generally, any treatment that can reasonably be expected to reverse illness or restore health is justified, whereas withholding that treatment requires specific ethical justification. Conversely, if treatment will definitely not reverse a disease process or restore health, then the decision to initiate such treatment may not be justified and may be unethical. Until recently, nearly all patients in the United States—even those who were clearly about to die—received maximal treatment (sometimes contrary to the patient’s or family’s wishes) for fear of the possible legal repercussions of withholding treatment. “Heroic” measures such as chest compressions, drug resuscitation, and mechanical ventilation were continued until the patient died. These complex decisions must involve the patient (or guardian) and the family and must be consistent with hospital policies and state and federal law.
Fortunately, legal guidelines for arriving at these decisions are available in nearly all states. Although laws vary from state to state, they tend to be similar. The greatest conundrums relate to withholding treatment and discontinuing artificial life-support systems. Competent patients (ie, individuals who have the capacity to understand and make medical decisions) have the right to refuse treatment and the right to have life-support machines or devices turned off (or not initiated) if and when they so request. Most states allow competent individuals to prepare an advance directive, usually either a living will or a durable power of attorney for health care, to prevent needless prolongation of life if they become incompetent (eg, severe mental disability, vegetative state, or irreversible coma). Withholding treatment or discontinuing life support from patients who do not have advance directives or cannot provide their own consent requires permission of the spouse, guardian, next of kin, or an individual to whom the patient has given power of attorney for health care. In some cases, clarification from the courts may be necessary. “Do Not Resuscitate” (DNR) or “Allow Natural Death” (AND) orders have been upheld by the courts for patients in whom resuscitation offers no hope of curing or reversing the disease process responsible for imminent death.
Artificial support of ventilation and circulation complicates legal definitions of death. Until recently, most states required only a determination by a physician that irreversible cessation of ventilatory and circulatory function had occurred. All states have added the concept of brain death to that definition, while some states recognize religious exemptions. In New Jersey, for example, physicians cannot declare brain death “if it would violate the personal religious beliefs of the individual.” In addition, although brain death can be established in a pregnant woman, the issue of whether life support can be withdrawn remains subject to both ethical and legal debate. There have been a number of cases of women giving birth to a viable baby weeks or months after having been declared brain dead. These cases involve issues of maternal rights, “fetal rights,” and paternal rights and have yet to be resolved.
Brain death is defined as irreversible cessation of all brain function. Spinal cord function below C1 may still be present. Establishing brain death relieves the burden on families of unjustifiable hope and prolonged anxiety; it also prevents waste of medical resources, and potentially allows the retrieval of organs for transplantation.
Brain death criteria can be applied only in the absence of hypothermia, hypotension, metabolic or endocrine abnormalities, neuromuscular blockers, and drugs known to depress brain function. A toxicology screen is required if sufficient time since admission (at least 3 days) has not elapsed to exclude a drug effect. Moreover, the patient should be observed long enough to establish with reasonable certainty the irreversible nature of the injury. Generally accepted clinical criteria for brain death include the following:
Coma
Absent motor activity, including no decerebrate or decorticate posturing; spinal cord reflexes may be preserved in some patients
Absent brainstem reflexes, including no pupillary, corneal, vestibuloocular (caloric), or gag (or cough) reflexes
Absence of ventilatory effort, with the arterial CO2 tension at least 60 mm Hg or 20 mm Hg above the pretest level.
Repeating the examination (not less than 2 h apart) is optional. In the United States the required number of physician observers varies by state (Florida requires two), as does the level of expertise (Virginia requires a neurologist or neurosurgeon to make the determination). The apnea test should be reserved for last because of its detrimental effects on intracranial pressure. Confirmatory test findings that may be helpful but are not required include an isoelectric electroencephalogram, absence of brainstem auditory evoked potentials, and absence of cerebral perfusion as documented by angiographic, transcranial Doppler, or radioisotopic studies.
Respiratory Care
Respiratory care refers both to the delivery of pulmonary therapy and diagnostic tests and to the allied health profession that has become an integral part of cardiopulmonary diagnostics and critical care. Respiratory therapists’ scope of practice encompasses medical gas therapy, delivery of aerosolized medications, airway management, mechanical ventilation, positive airway pressure therapy, critical care monitoring, cardiopulmonary rehabilitation, and the application of various techniques collectively termed chest physiotherapy. The latter includes administering aerosols, clearing pulmonary secretions, reexpansion of atelectatic lung, and preserving normal lung function postoperatively or during illness. Diagnostic services may include pulmonary function testing, arterial blood gas analysis, electrocardiography testing, and evaluation of sleep-disordered breathing. The majority of respiratory care procedures are based on clinical practice guidelines developed by the American Association for Respiratory Care using best practice/evidence-based medicine criteria.
The therapeutic medical gases include oxygen at ambient or hyperbaric pressure, helium-oxygen mixtures (heliox), and nitric oxide. Oxygen is made available in high-pressure cylinders, via pipeline systems, from oxygen concentrators, as well as in liquid form. Heliox is occasionally used to partially relieve the increased work of breathing due to partial upper airway obstruction. Nitric oxide is administered as a direct, selective pulmonary vasodilator.
The primary goal of oxygen therapy is to prevent or correct hypoxemia or tissue hypoxia. Table 57-1 identifies classic categories of hypoxia. Oxygen therapy alone may not correct either hypoxemia or hypoxia. Continuous positive airway pressure (CPAP) or positive end-expiratory pressure (PEEP) may be required to recruit collapsed alveoli. Patients with profound hypercapnia may require ventilatory assistance. High concentrations of oxygen may be indicated for conditions requiring removal of entrapped gas (eg, nitrogen) from body cavities or vessels. The short-term inhalation of increased concentrations of oxygen is relatively free of complications.
Hypoxia | Pathophysiologic Category | Clinical Example |
---|---|---|
Hypoxic hypoxia | ↓ PBarom or ↓ Fio2 (<0.21) Alveolar hypoventilation Pulmonary diffusion defect Pulmonary R → L shunt | Altitude, O2 equipment error Drug overdose, COPD exacerbation Asthma, pulmonary emboli Atelectasis, cyanotic congenital heart disease |
Circulatory hypoxia | Reduced cardiac output Microvascular dysfunction | Severe heart failure, dehydration Sepsis, SIRS |
Hemic hypoxia | Reduced hemoglobin content Reduced hemoglobin function | Anemias Carboxyhemoglobinemia, methemoglobinemia |
Demand hypoxia Histotoxic hypoxia | ↑ Oxygen consumption Inability of cells to utilize oxygen | Fever, seizures Cyanide toxicity, ↑TNF, late sepsis |
Supplemental oxygen is indicated for adults, children, and infants (older than 1 month) when Pao2 is less than 60 mm Hg (8 kPa) or Sao2 or Spo2 is less than 90% while at rest breathing room air. In neonates, therapy is recommended if Pao2 is less than 50 mm Hg (6.7 kPa) or Sao2 is less than 88% (or capillary Po2 is less than 40 mm Hg [5.3 kPa]). Therapy may be indicated for patients when clinicians suspect (rather than measure) hypoxemia or hypoxia based on a medical history and physical examination. Patients with myocardial infarction, cardiogenic pulmonary edema, acute lung injury, acute respiratory distress syndrome (ARDS), pulmonary fibrosis, cyanide poisoning, or carbon monoxide inhalation all require supplemental oxygen. Supplemental oxygen is given during the perioperative period because general anesthesia commonly causes a decrease in Pao2 secondary to increased pulmonary ventilation/perfusion mismatching and decreased functional residual capacity (FRC). Supplemental oxygen should be provided before procedures such as tracheal suctioning or bronchoscopy, which commonly cause arterial desaturation. There is evidence that supplemental oxygen is effective in prolonging survival of patients with chronic obstructive pulmonary disease (COPD) whose resting Pao2 is lower than 60 mm Hg at sea level. Supplemental oxygen therapy also appears to have a mild beneficial effect on the mean pulmonary arterial pressure and subjective indices of patients’ dyspnea.
Oxygen given alone or in a gas can be mixed with air as a partial supplement to patients’ tidal or minute volume or serve as the entire source of the inspired volume. This approach provides the basis for classifying devices or systems according to their ability to provide adequate flow levels and a range of fraction of inspired oxygen (Fio2). Other considerations in selecting therapy include patient compliance, the presence and type of artificial airway, and the need for humidification or an aerosol delivery system.
Oxygen (usually 100%) is supplied at a fixed flow that is only a portion of inspired gas. Such devices are usually intended for patients with stable breathing patterns. As ventilatory demands change, variable amounts of room air will dilute the oxygen flow. Low-flow systems are adequate for patients with
- Minute ventilation less than ˜8-10 L/min
- Breathing frequencies less than ˜20 breaths/min
- Tidal volumes (VT) less than ˜0.8 L
- Normal inspiratory flow (10-30 L/min).
Inspired gas at a preset Fio2 is supplied continuously at high flow or by providing a sufficiently large reservoir of premixed gas. Ideally, the delivered Fio2 is not affected by variations in ventilatory level or breathing pattern. Profoundly dyspneic and hypoxemic patients may need flows of 100% oxygen in excess of 100 L/min. High-flow systems are indicated for patients who require
- Consistent Fio2
- Large inspiratory flows of gas (>40 L/min).
The nasal cannula is available as either a blind-ended soft plastic tube with an over-the-ear head-elastic or dual-flow with under-the-chin lariat adjustment. Sizes appropriate for adults, children, and infants are available. Cannulas are connected to flowmeters with small-bore tubing and can rapidly be placed on most patients. The tension of attachment should be firm yet comfortable enough to avoid pressure sores on the ears, cheeks, and nose. Patients receiving long-term oxygen therapy most commonly use a nasal cannula. The appliance is usually well tolerated, allowing unencumbered speech, eating, and drinking. Cannulas can be combined with spectacle frames for convenience or to improve acceptance by improving cosmesis. Oxygen-conserving cannulas equipped with inlet reservoirs are available for patients receiving long-term oxygen. Since oxygen flows continuously, approximately 80% of the gas is wasted during expiration. There are valved reservoir devices that permit storage of incoming oxygen until inspiration occurs.
Device/System | Oxygen Flow Rate (L/min) | Fio2 Range |
---|---|---|
Nasal cannula | 1 | 0.21-0.24 |
2 | 0.23-0.28 | |
3 | 0.27-0.34 | |
4 | 0.31-0.38 | |
5-6 | 0.32-0.44 | |
Simple mask | 5-6 | 0.30-0.45 |
7-8 | 0.40-0.60 | |
Mask with reservoir | 5 | 0.35-0.50 |
Partial rebreathing mask-bag | 7 | 0.35-0.75 |
15 | 0.65-1.00 | |
Nonrebreathing mask-bag | 7-15 | 0.40-1.00 |
Venturi mask and jet nebulizer | 4-6 (total flow = 15) | 0.24 |
4-6 (total flow = 45) | 0.28 | |
8-10 (total flow = 45) | 0.35 | |
8-10 (total flow = 33) | 0.40 | |
8-12 (total flow = 33) | 0.50 |
The actual Fio2 delivered to adults with nasal cannulas is determined by oxygen flow, nasopharyngeal volume, and the patient’s inspiratory flow (which depends both on Vt and inspiratory time). Oxygen from the cannula can fill the nasopharynx after exhalation, yet with inspiration, oxygen and entrained air are drawn into the trachea. The inspired percent oxygen increases by approximately 1-2% (above 21%) per liter of oxygen flow with quiet breathing in adults. Cannulas can be expected to provide inspired oxygen concentrations up to 30-35% with normal breathing and oxygen flows of 3-4 L/min. However, levels of 40-50% can be attained with oxygen flows of greater than 10 L/min for short periods. Flows greater than 5 L/min are poorly tolerated because of the discomfort of gas jetting into the nasal cavity and because of drying and crusting of the nasal mucosa.
Data from “normal-breathing subjects” may not be accurate for acutely ill tachypneic patients. Increasing Vt and reducing inspiratory time will dilute the small flow of oxygen. Different proportions of mouth-only versus nose-only breathing and varied inspiratory flow can alter Fio2 by up to 40%. In clinical practice, flow should be titrated according to vital signs, pulse oximetry, and arterial blood gas measurements. Some patients with COPD tend to hypoventilate with even modest oxygen flows, yet are hypoxemic on room air. They may do well with cannula flows of less than 1-2 L/min.
Pediatric-sized nasal cannulas are available. Special cannulas allow babies to nurse and produce less trauma of the face and nose than oxygen masks. Because of the inherently reduced minute ventilation of infants, flow requirements to the cannula must be proportionately reduced. This generally requires a pressure-compensated flowmeter accurate at delivering oxygen flows in the less than 1-3 L/min range. Hypopharyngeal oxygen sampling from infants breathing with cannulas has demonstrated mean Fio2 of 0.35, 0.45, 0.6, and 0.68 with flows of 0.25, 0.5, 0.75, and 1.0 L/min, respectively.
The nasal mask is a hybrid of the nasal cannula and a face mask. It can be applied to the face by either an over-the-ear lariat or a headband strap. The lower edge of the mask’s flanges rests on the upper lip, surrounding the external nose. Nasal masks have been shown to provide supplemental oxygen equivalent to the nasal cannula under low-flow conditions for adult patients. The primary advantage of the nasal mask over nasal cannulas appears to be patient comfort. The nasal mask does not produce sores around the external nares and dry oxygen is not “jetted” into the nasal cavity. The nasal mask should be considered if it improves patient comfort and compliance.
The “simple” or oxygen mask is a disposable lightweight plastic device that covers both nose and mouth. It has no reservoir bag. Masks are fastened to the patient’s face by adjustment of an elastic headband; some manufacturers provide a malleable metal nose-bridge adjustment device. The seal is rarely complete: usually there is “inboard” leaking. Thus, patients receive a mixture of oxygen and secondarily entrained room air. This varies depending on the size of the leak, oxygen flow, and breathing pattern. Some brands of the simple mask connect tubing to a standard tapered fitting; others have a small room air-entrainment hole at the connection.
The body of the mask functions as a reservoir for both oxygen and expired carbon dioxide. A minimum oxygen flow of approximately 5 L/min is applied to the mask to limit rebreathing and the resulting increased respiratory work. Wearing any mask appliance for long periods of time is uncomfortable. Speech is muffled and drinking and eating are difficult.
It is difficult to predict delivered Fio2 at specific oxygen flow rates. During normal breathing, it is reasonable to expect an Fio2 of 0.3-0.6 with flows of 5-10 L/min, respectively. Oxygen levels can be increased with smaller VT or slower breathing rates. With higher flows and ideal conditions, Fio2 may approach 0.7 or 0.8.
Masks lacking oxygen reservoirs may be best suited for patients who require concentrations of oxygen greater than cannulas provide, yet need oxygen therapy for fairly short periods of time. Examples would include medical transport or therapy in the postanesthesia care unit or emergency department. It is not the device of choice for patients with severe respiratory disease who are profoundly hypoxemic, tachypneic, or unable to protect their airway from aspiration.
Incorporating a gas reservoir is a logical adaptation to the simple mask. Two types of reservoir mask are commonly used: the partial rebreathing mask and the nonrebreathing mask. Both are disposable, lightweight, transparent plastic under-the-chin reservoirs. The difference between the two relates to use of valves on the mask and between the mask and the bag reservoir. Mask reservoirs commonly hold approximately 600 mL or less of gas volume. The phrase “partial rebreather” is used because “part” of the patient’s expired VT refills the bag. Usually that gas is largely dead space that should not result in significant rebreathing of carbon dioxide.
The nonrebreather uses the same basic system as the partial rebreather but incorporates flap-type valves between the bag and mask and on at least one of the mask’s exhalation ports. Inboard leaking is common, and room air will enter during brisk inspiratory flows, even when the bag contains gas. The lack of a complete facial seal and a relatively small reservoir influence the delivered oxygen concentration. The key factor in successful application of the masks is to use a sufficiently high flow of oxygen, so that the reservoir bag is at least partially full during inspiration. Typical minimum flows of oxygen are 10-15 L/min. Well-fitting partial rebreathing masks provide a range of FIO2 from 0.35 to 0.60 with oxygen flows up to 10 L/min. With inlet flows of 15 L/min or more and ideal breathing conditions, FIO2 may approach 1.0. Either style of mask is indicated for patients suspected of having significant hypoxemia, with relatively normal spontaneous minute ventilation. Such patients may include victims of trauma, myocardial infarction, or carbon monoxide exposure. Profoundly dyspneic patients with gasping respiration may be served by a fixed-performance, high-flow oxygen system.
The basic design follows that of the nonrebreathing reservoir mask but with more “capable” components. Self-inflating bags consist of a roughly 1.5 L bladder, usually with an oxygen inlet reservoir. Anesthesia bags are 1-, 2-, or 3-L non-self-inflating reservoirs with a tailpiece gas inlet. Masks are designed to provide a comfortable leak-free seal for manual ventilation. The inspiratory/expiratory valve systems may vary. The flow to the reservoir should be kept high so that the bags do not deflate substantially. When using an anesthesia bag, operators may frequently have to adjust the oxygen flow and exhaust valve to respond to changing breathing patterns or demands, particularly when maintaining a complete seal between the mask and face is difficult.
The most common systems for disposable and permanent self-inflating resuscitation bags use a unidirectional gas flow. Although these devices offer the potential for a constant FIO2 greater than 0.9, tailpiece inlet valves will not open for a spontaneously breathing patient. Opening the valves requires negative pressure bag recoil after compression. If this situation is not recognized, clinicians might be misled into thinking the patient is receiving a specific concentration of oxygen when this is not the case.
There are limits to the ability of each system to maintain its fixed-performance characteristics. Delivered FIO2 can approach 1.0 with either anesthesia or self-inflating bags. Spontaneously breathing patients are allowed to breathe only the contents of the system if the mask seal is tight and the reservoir is adequately maintained.
Failure to maintain an adequate oxygen supply in the reservoir and inlet flow is a concern. The spring-loaded valve of anesthesia bags must be adjusted to prevent overdistention of the bag. Self-inflating bags look the same whether or not oxygen flow to the unit is adequate, and they will entrain room air into the bag, thus lowering the delivered FIO2.
The gas delivery approach with air-entraining masks is different than with an oxygen reservoir. The goal is to create an open system with high flow about the nose and mouth, with a fixed FIO2. Oxygen is directed by small-bore tubing to a mixing jet; the final oxygen concentration depends on the ratio of air drawn in through entrainment ports. Manufacturers have developed both fixed and adjustable entrainment selections over an FIO2 range. Most provide instructions for the operator to set a minimum flow of oxygen. Table 57-3 identifies total flow at various inlet flows and FIO2.
Despite the high-flow concept, FIO2 can vary up to 6% from the anticipated setting. The air-entraining masks are a logical choice for patients who require greater FIO2 than can be provided by devices such as the nasal cannula. Patients with COPD who tend to hypoventilate with a moderate FIO2 are candidates for the Venturi mask. Clinicians providing oxygen therapy with Venturi masks should be aware of the previously mentioned problems involving the mask itself. FIO2 can increase if the air entrainment ports are obstructed by the patient’s hands, bed sheets, or water condensate. Clinicians should encourage the patient and caregivers to keep the mask on the face continuously. Interruption of oxygen is a serious problem in unstable patients with hypoxemia and or hypercarbia.
Direct analysis of the FIO2 during air-entrainment mask breathing is difficult to perform accurately. Arterial blood gas analysis and the patient’s respiratory rate should guide clinicians as to whether the patient’s demands are being met by the mask’s flow. If that occurs, then inlet oxygen flows may need to be increased or an alternate device selected.
Large-volume, high-output or “all-purpose” nebulizers have been used in respiratory care for many years to provide mist therapy with some control of the FIO2. These units are commonly placed on patients following extubation for their aerosol-producing properties. Like the air-entraining masks, nebulizers use a pneumatic jet and an adjustable orifice to vary entrained air for varying FIO2 levels. Many commercial devices have an inlet orifice diameter that maximally allows only 15 L/min when the source pressure is 50 psi. This means that on the 100% setting (no air entrainment) output flow is only 15 L/min. Only patients breathing at slow rates and small VT will receive 100% oxygen. This problem has been addressed by the development of high-flow, high-FIO2 nebulizers. For more common applications that use an FIO2 of 0.3-0.5, room air is entrained, reducing the FIO2 and increasing the total flow output to 40-50 L/min.
Knowledge of the air/oxygen ratio and the input flow rate of oxygen allows the total outflow to be calculated. Nebulizer systems can be applied to the patient with many different devices, including aerosol, tracheostomy dome/collar, face tent, and Τ-piece adapter. These appliances can all be attached via large-bore tubing to the nebulizer. This open system freely vents inspiratory and expiratory gases around the patient’s face or out a distal port of a Τ-piece adapter. Unfortunately, the lack of any valves allows patients to secondarily entrain room air. It is common practice to use either a reservoir bag before the Τ-piece or a reservoir tube on the distal side of the Τ-piece to provide a larger volume of gas than that coming from the nebulizer. A typical concern of those applying air-entrainment aerosol therapy with controlled oxygen concentration is whether the system will provide adequate flow. Clinicians should observe the mist like a tracer to determine adequacy of flow. When a Τ-piece is used and the visible mist (exiting the distal port) disappears during inspiration, the flow is inadequate.
Another concern in clinical practice is that excess water in the tubing collects and can obstruct gas flow completely or can offer increased resistance to flow. The latter may increase the FIO2 above the desired setting. Other complications include bronchospasm or laryngospasm in some patients as a consequence of airway irritation from sterile water droplets (condensate of the aerosol). In such circumstances, a heated (nonaerosol) humidification system should be substituted.
Dual air-oxygen flowmeters and air-oxygen blenders are commonly used for oxygen administration as well as freestanding continuous positive airway pressure (CPAP) and “add-on” ventilator systems. These systems differ from the air-entraining nebulizers, as their total output flows do not diminish at FIO2 greater than 0.4. With these high-flow systems, the total flow to the patient and FIO2 can be set independently to meet patient needs. This can be done using a large reservoir bag or constant flows in the range of 50 to more than 100 L/min. Clinicians can use a variety of appliances with these systems, including aerosol masks, face tents, or well-fitted nonrebreathing system masks with air-oxygen blenders. Face-sealing mask systems can also be constructed with a reservoir bag and a safety valve to allow breathing if the blender fails. The high flows of gas require use of heated humidifiers of the type commonly used on mechanical ventilators. Humidification offers an advantage for patients with reactive airways. Because of the high flows, such systems are used to apply CPAP or BIPAP for spontaneously breathing patients.
Although many of the devices previously described have pediatric-sized options, many infants and neonates will not tolerate facial appliances. Oxygen hoods cover only the head, allowing access to the child’s lower body while still permitting use of a standard incubator or radiant warmer. The hood is ideal for relatively short-term oxygen therapy for newborns and inactive infants. However, for mobile infants requiring longer term therapy, the nasal cannula, face mask, or full-bed enclosure allow for greater mobility.
Normally, oxygen and air are premixed by an air-oxygen blender and passed through a heated humidifier. Nebulizers should be avoided. Most pneumatic jet-type nebulizers create noise levels (>65 dB) that may cause newborn hearing loss, and cold gas can induce an increase in oxygen consumption. Hoods come in different sizes. Some are simple Plexiglas boxes; others have elaborate systems for sealing the neck opening. There is no attempt to completely seal the system, as a constant flow of gas is needed to remove carbon dioxide (minimum flow >7 L/min). Hood inlet flows of 10-15 L/min are adequate for a majority of patients.
Helium-oxygen (heliox) mixtures have a notable, yet limited clinical role. In addition to its uses in industry and deep-sea diving, heliox has a number of medical applications. Helium is premixed with oxygen in several standard blends. The most popular mixture is 79%/21% helium-oxygen, which has a density that is 40% that of pure oxygen. Helium-oxygen mixtures are available in large-sized compressed gas cylinders.
In anesthetic practice, pressures needed to ventilate patients with small-diameter tracheal tubes can be substantially reduced when the 79%/21% mixture is used. Heliox can provide patients with upper airway-obstructing lesions (eg, subglottic edema, foreign bodies, and tracheal tumors) with relief from acute distress until more definitive care can be delivered. The evidence is less convincing in treating lower airway obstruction from COPD or acute asthma. Helium mixtures may also be used as the driving gas for small-volume nebulizers in bronchodilator therapy for asthma. However, with heliox, the nebulizer flow needs to be increased to 11 L/min versus the usual 6-8 L/min with oxygen. Patients’ work of breathing can be reduced with heliox as compared to a conventional oxygen/nitrogen gas mixture.
Hyperbaric oxygen therapy uses a pressurized chamber to expose the patient to oxygen tensions exceeding ambient barometric pressure (at sea level the ambient pressure is 760 mm Hg). With a one-person hyperbaric chamber, 100% oxygen is usually used to pressurize the chamber. Larger chambers allow for the simultaneous treatment of multiple patients and for the presence of medical personnel in the chamber with patients. Multiplace chambers use air to pressurize the chamber, whereas patients receive 100% oxygen by mask, hood, or tracheal tube. Common indications for hyperbaric oxygen include decompression sickness (the “bends”), certain forms of gas embolism, gas gangrene, carbon monoxide poisoning, and treatment of certain wounds.
Oxygen therapy can result in both respiratory and nonrespiratory toxicity. Important factors include patient susceptibility, the FIO2, and duration of therapy.
This complication is primarily seen in patients with COPD who have chronic CO2 retention. These patients develop an altered respiratory drive that becomes at least partly dependent on the maintenance of relative hypoxemia. Elevation of arterial oxygen tension to “normal” can therefore cause severe hypoventilation in these patients. Conversely, stable, spontaneously breathing patients with profound hypercarbia (PaCO2 > 80 mm Hg) who are being supported with supplemental oxygen should not have supplemental oxygen discontinued, even for short intervals. Oxygen therapy can be indirectly hazardous for patients being monitored with pulse oximetry while receiving opioids for pain. Hypoventilation as a consequence of opioids may fail to cause worrisome change in oxygen saturation, despite respiratory rates as infrequent as 2 per minute, delaying the diagnosis.
High concentrations of oxygen can cause pulmonary atelectasis in areas of low ratios. As nitrogen is “washed out” of the lungs, the lowered gas tension in pulmonary capillary blood results in increased uptake of alveolar gas and absorption atelectasis. If the area remains perfused but nonventilated, the resulting intrapulmonary shunt can lead to progressive widening of the alveolar-to-arterial (A-a) gradient.
Prolonged high concentrations of oxygen may damage the lungs. Toxicity is dependent both on the partial pressure of oxygen in the inspired gas and the duration of exposure. Alveolar rather than arterial oxygen tension is most important in the development of oxygen toxicity. Although 100% oxygen for up to 10-20 h is generally considered safe, concentrations greater than 50-60% are undesirable for longer periods as they may lead to pulmonary toxicity.
Molecular oxygen (O2) is unusual in that each atom has unpaired electrons. This gives the molecule the paramagnetic property that allows precise measurements of oxygen concentration. Notably, internal rearrangement of these electrons or their interaction with other atoms (iron) or molecules (xanthine) can produce potentially toxic chemical species. Oxygen toxicity is thought to be due to intracellular generation of highly reactive O2 metabolites (free radicals) such as superoxide and activated hydroxyl ions, singlet O2, and hydrogen peroxide. A high concentration of O2 increases the likelihood of generating toxic species. These metabolites are cytotoxic because they readily react with cellular DNA, sulfhydryl proteins, and lipids. Two cellular enzymes, superoxide dismutase and catalase, protect against toxicity by sequentially converting superoxide first to hydrogen peroxide and then to water. Additional protection may be provided by antioxidants and free radical scavengers; however, clinical evidence supporting the use of these agents in preventing pulmonary toxicity is lacking.
In experimental animals oxygen-mediated injury of the alveolar-capillary membrane produces a syndrome that is pathologically and clinically indistinguishable from ARDS. Tracheobronchitis may also be present initially in some patients. Pulmonary O2 toxicity in newborn infants is manifested as bronchopulmonary dysplasia.
Retinopathy of prematurity (ROP), formerly termed retrolental fibroplasia, is a neovascular retinal disorder that develops in 84% of premature survivors born at less than 28 weeks’ gestation. ROP may include disorganized vascular proliferation and fibrosis and may lead to retinal detachment and blindness. ROP resolves in approximately 80% of these cases without visual loss from retinal detachments or scars. ROP was very common in the 1940s-1950s when unmonitored high (>0.5 FIO2) oxygen was often administered to premature infants. However, it is now known that hyperoxia and hypoxia are risk factors, but not the primary causes of ROP. Neonates’ risk of ROP increases with low birth weight and complexity of comorbidities (eg, sepsis). In contrast to pulmonary toxicity, ROP correlates better with arterial than with alveolar O2 tension. The recommended arterial concentrations for premature infants receiving oxygen are 50-80 mm Hg (6.6-10.6 kPa). If an infant requires arterial O2 saturations of 96%-99% for cardiopulmonary reasons, fear about causing or worsening ROP is not a reason to withhold the oxygen.
The high inspired O2 tensions associated with hyperbaric O2 therapy greatly accelerate O2 toxicity. The risk and expected degree of toxicity are directly related to the pressures used as well as the duration of exposure. Prolonged exposure to O2 partial pressures in excess of 0.5 atmospheres can cause pulmonary O2 toxicity. This may present initially with retrosternal burning, cough, and chest tightness and will result in progressive impairment of pulmonary function with continued exposure. Patients exposed to O2 at 2 atmospheres or greater are also at risk for central nervous system toxicity that may be expressed as behavior changes, nausea, vertigo, muscular twitching, or convulsions.
Oxygen vigorously supports combustion. The potential for oxygen enriched gas mixtures to promote fires and explosions is discussed in Chapter 2.
Despite early intervention and appropriate respiratory care, patients with critical illness will often require mechanical ventilation. Mechanical ventilation can replace or supplement normal spontaneous ventilation. In most instances, the problem is primarily that of impaired CO2 elimination (ventilatory failure). In other instances, mechanical ventilation may be used as an adjunct (usually to positive-pressure therapy; see below) in the treatment of hypoxemia. The decision to initiate mechanical ventilation is made on clinical grounds, but certain parameters have been suggested as guidelines (Table 57-4).
Criterion | Measurement |
---|---|
Direct measurement | |
Arterial oxygen tension Arterial CO2 tension | <50 mm Hg on room air >50 mm Hg in the absence of metabolic alkalosis |
Derived indices | |
Pao2/Fio2 ratio | <300 mm Hg |
PA-ao2 gradient | >350 mm Hg |
Vd/Vt | >0.6 |
Clinical indices | |
Respiratory rate | >35 breaths/min |
Mechanical indices | |
Tidal volume | <5 mL/kg |
Vital capacity | <15 mL/kg |
Maximum inspiratory force | > −25 cm H2O (eg, -15 cm H2O) |
Of the two available techniques, positive-pressure ventilation and negative-pressure ventilation, the former has much wider applications and is almost universally used. Although negative-pressure ventilation does not require tracheal intubation, it cannot overcome substantial increases in airway resistance or decreases in pulmonary compliance, and it also limits access to the patient.
During positive-pressure ventilation, lung inflation is achieved by periodically applying positive pressure to the upper airway through a tight-fitting mask (noninvasive mechanical ventilation) or through a tracheal or tracheostomy tube. Increased airway resistance and decreased lung compliance can be overcome by manipulating inspiratory gas flow and pressure. The major disadvantages of positive-pressure ventilation are altered ventilation-to-perfusion relationships, potentially adverse circulatory effects, and risk of pulmonary barotrauma and volutrauma. Positive-pressure ventilation increases physiological dead space because gas flow is preferentially directed to the more compliant, nondependent areas of the lungs, whereas blood flow (influenced by gravity) favors dependent areas. Reductions in cardiac output are primarily due to impaired venous return to the heart from increased intrathoracic pressure. Barotrauma is closely related to repetitive high peak inflation pressures and underlying lung disease, whereas volutrauma is related to the repetitive collapse and reexpansion of alveoli.
Positive-pressure ventilators periodically create a pressure gradient between the machine circuit and alveoli that results in inspiratory gas flow. Exhalation occurs passively. Ventilators and their control mechanisms can be powered pneumatically (by a pressurized gas source), electrically, or by both mechanisms. Gas flow is either derived directly from the pressurized gas source or produced by the action of a rotary or linear piston. This gas flow then either goes directly to the patient (single-circuit system) or, as commonly occurs with operating room ventilators, compresses a reservoir bag or bellows that is part of the patient circuit (double-circuit system).
All ventilators have four phases: inspiration, the changeover from inspiration to expiration, expiration, and the changeover from expiration to inspiration (see Chapter 4). These phases are defined by VT, ventilatory rate, inspiratory time, inspiratory gas flow, and expiratory time.
The complexity of modern ventilators defies simple classification. Incorporation of microprocessor technology into the newest generation of ventilators has further complicated this task. Nonetheless, ventilators are most commonly classified according to their inspiratory phase characteristics and their method of cycling from inspiration to expiration.
Most modern ventilators behave like flow generators. Constant flow generators deliver a constant inspiratory gas flow regardless of airway circuit pressure. Constant flow is produced by the use of either a solenoid (on-off) valve with a high-pressure gas source (5-50 psi) or via a gas injector (Venturi) with a lower-pressure source. Machines with high-pressure gas sources allow inspiratory gas flow to remain constant despite large changes in airway resistance or pulmonary compliance. The performance of ventilators with gas injectors varies more with airway pressure. Nonconstant flow generators consistently vary inspiratory flow with each inspiratory cycle (such as by a rotary piston); a sine wave pattern of flow is typical.
Constant-pressure generators maintain airway pressure constant throughout inspiration and irrespective of inspiratory gas flow. Gas flow ceases when airway pressure equals the set inspiratory pressure. Pressure generators typically operate at low gas pressures (just above peak inspiratory pressure).
Time-cycled ventilators cycle to the expiratory phase once a predetermined interval elapses from the start of inspiration. VT is the product of the set inspiratory time and inspiratory flow rate. Time-cycled ventilators are commonly used for neonates and in the operating room.
Volume-cycled ventilators terminate inspiration when a preselected volume is delivered. Many adult ventilators are volume cycled but also have secondary limits on inspiratory pressure to guard against pulmonary barotrauma. If inspiratory pressure exceeds the pressure limit, the machine cycles into expiration even if the selected volume has not been delivered.
Properly functioning volume-cycled ventilators do not deliver the set volume to the patient. A percentage of the set VT is always lost due to expansion of the breathing circuit during inspiration. Circuit compliance is usually about 3-5 mL/cm H2O; thus, if a pressure of 30 cm H2O is generated during inspiration, 90-150 mL of the set VT is lost to the circuit. Loss of VT to the breathing circuit is therefore inversely related to lung compliance. For accurate measurement of the exhaled VT, the spirometer must be placed at the tracheal tube rather than the exhalation valve of the ventilator.
Pressure-cycled ventilators cycle into the expiratory phase when airway pressure reaches a predetermined level. VT and inspiratory time vary, being related to airway resistance and pulmonary and circuit compliance. A significant leak in the patient circuit can prevent the necessary rise in circuit pressure and machine cycling. Conversely, an acute increase in airway resistance, or decrease in pulmonary compliance, or circuit compliance (kink) causes premature cycling and decreases the delivered VT. Pressure-cycled ventilators have been most often used for short-term indications (transport).
Flow-cycled ventilators have pressure and flow sensors that allow the ventilator to monitor inspiratory flow at a preselected fixed inspiratory pressure; when this flow reaches a predetermined level (usually 25% of the initial peak mechanical inspiratory flow rate), the ventilator cycles from inspiration into expiration (see the sections on Pressure Support and Pressure Control Ventilation).
These versatile machines can be set to function in any one of a variety of inspiratory flow and cycling patterns. The microprocessor allows closed-loop control over the ventilator’s performance characteristics. Microprocessor-controlled ventilators are the norm in modern critical care units and on newer anesthesia machines.
Ventilatory mode is defined by the method by which the ventilator cycles from expiration to inspiration as well as whether the patient is able to breathe spontaneously (Table 57-5 and Figure 57-1). Most modern ventilators are capable of multiple ventilatory modes, and some (microprocessor-controlled ventilators) can combine modes simultaneously. Typical ventilatory modes are regulated to deliver a defined VT or a defined maximal inspiratory pressure. Modern ventilators can provide for breaths that are volume-controlled (machine-initiated inspiration stops when the set volume is delivered), volume-assisted (patient-initiated inspiration stops when the set volume is delivered), pressure-controlled (machine-initiated inspiration at a mandatory inspiratory pressure stops after a defined time has elapsed), pressure-assisted (patient-initiated inspiration at a mandatory inspiratory pressure stops after a defined time has elapsed), or pressure-supported (patient-initiated inspiration continues at a mandatory inspiratory pressure until the inspiratory flow declines to a defined value).
In this mode, the ventilator cycles from expiration to inspiration after a fixed time interval. The interval determines the ventilatory rate. Typical settings on this mode provide a fixed VT and fixed rate (and, therefore, minute ventilation) regardless of patient effort, because the patient cannot breathe spontaneously. Settings to limit inspiratory pressure guard against pulmonary barotrauma, and indeed CMV can be provided in a pressure-limited (rather than volume-limited) way. Controlled ventilation is best reserved for patients capable of little or no ventilatory effort. Awake patients with active ventilatory effort require sedation, possibly with muscle paralysis.
Incorporation of a pressure sensor in the breathing circuit of AC ventilators permits the patient’s inspiratory effort to be used to trigger inspiration. A sensitivity control allows selection of the inspiratory effort required. The ventilator can be set for a fixed ventilatory rate, but each patient effort of sufficient magnitude will trigger the set VT. If spontaneous inspiratory efforts are not detected, the machine functions as if in the control mode. Most often, AC ventilation is used in a volume-limited format, but it can also be provided in a pressure-limited way (see below).
IMV allows spontaneous ventilation while the patient is on the ventilator. A selected number of mechanical breaths (with fixed VT) is given to supplement spontaneous breathing. At high mandatory rates (10-12 breaths/min), IMV essentially provides all of the patient’s ventilation; at low rates (1-2 breaths/min), it provides minimal mechanical ventilation and allows the patient to breathe relatively independently. The VT and frequency of spontaneous breaths are determined by the patient’s ventilatory drive and muscle strength. The IMV rate can be adjusted to maintain a desired minute ventilation. IMV has found greatest use as a weaning technique.
Synchronized intermittent mandatory ventilation (SIMV) times the mechanical breath, whenever possible, to coincide with the beginning of a spontaneous effort. Proper synchronization prevents superimposing (stacking) a mechanical breath in the middle of a spontaneous breath, resulting in a very large VT
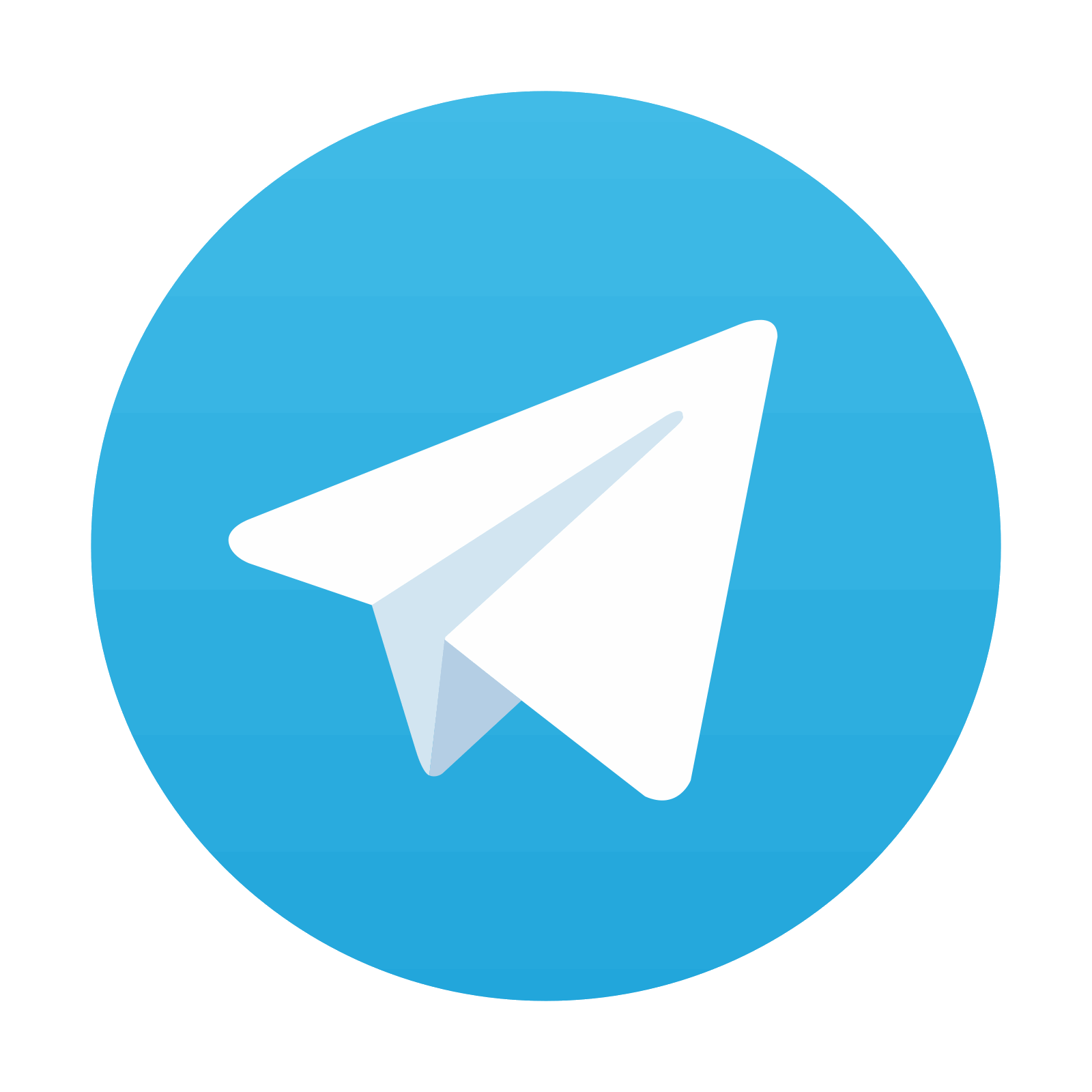
Stay updated, free articles. Join our Telegram channel
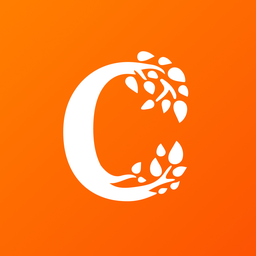
Full access? Get Clinical Tree
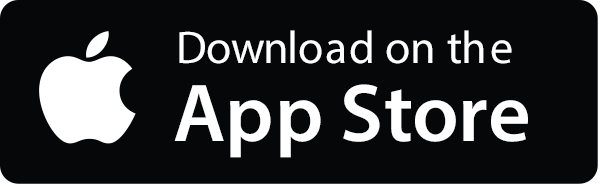
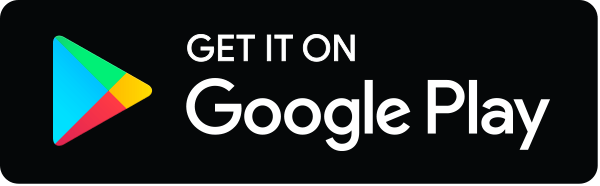
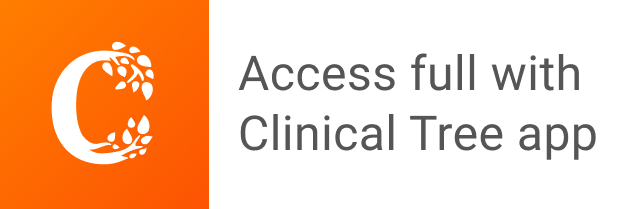