The clinical tool most commonly used to assess cardiac function is the surface electrocardiogram (ECG). The ECG records the sum of the electrical changes occurring within the myocardium. The electrophysiologic basis of cardiac function and the ECG are complex and are subject to alteration by numerous xenobiotics. Ion currents flowing through various ion channels are responsible for cardiac function. Electrophysiologic studies identify the functional types of membrane receptors and ion channels. Molecular genetic studies identify the gene coding for the key cardiac ion channels and elucidate the structural and physiologic relationships that lead to the toxic effects of many xenobiotics. These channels are critical for maintenance of the intracellular ion concentrations necessary for action potential development, impulse conduction throughout the heart, and myocyte contraction. This chapter will first review the individual ion channels and their currents, and then summarize their contribution and effects on the ECG.
The voltage-sensitive sodium channels are responsible for the initiation of depolarization of the myocardial membrane. All currently identified voltage-sensitive channels, including the sodium and calcium channels, have structures similar to the potassium channel assembly. The sodium channel gene encodes a single protein that contains 4 functional domains (D I to D IV). Each of these domains has the 6 membrane-spanning regions characteristic of the voltage-gated potassium channel and is structurally similar to the α subunit of the potassium channel. The single, large α subunit of the sodium channel assembles with regulatory β subunits to form the functional unit of the sodium channel (Fig. 15–1A). The best characterized of the sodium channels, the SCN5A gene–encoded α channel, is inactivated by xenobiotic interactions between the D III and the D IV domains to physically block the inner mouth of the sodium channel pore.29 Seven specific receptor sites are currently identified on the α subunit, with different xenobiotics binding at the specific sites: tetrodotoxin, saxitoxin, μ-conotoxin (site 1); aconitine, batrachotoxin, grayanotoxin, veratridine (site 2); α scorpion toxins, sea anemone toxins (site 3); β scorpion toxins, Chinese bird spider toxin (site 4); brevetoxins, ciguatoxins (site 5); δ-conotoxins (site 6); pyrethroids (site 7), and local anesthetics and related antidysrhythmics and antiepileptics binding at overlapping receptor sites.14,57,61,71
FIGURE 15–1.
Structure of the sodium and potassium channels. (A) The structure of the α subunit of the sodium channel. The protein molecule has 4 functional domains (D I–D IV) each analogous to one of the potassium channel α subunits. One of these molecules assembles with β subunits to form the membrane sodium channel. Sites 1–7 are shown with individual toxins known to bind at these sites. (B) The structure of the α-subunit of the voltage-gated potassium channel. The protein molecule has 6 membrane-spanning regions (1–6); the voltage-sensitive region is at 4 and the actual ion channel is located between regions 5 and 6, similar to the α sodium channel. Four of these α-subunits assemble with 4 β subunits to form the potassium channel complex.

Ion channels that change their conductance of current with changes in the transmembrane voltage potential are called rectifying channels. The voltage-sensitive potassium channels are categorized based on their speed of activation and their voltage response. They include the “delayed rectifier” potassium currents, particularly the IKr (rapidly activating) and the IKs (slowly activating) channels.43 Potassium channels form large multimolecular complexes with regulatory kinases, phosphatases, anchoring proteins, accessory subunits, and with each other.
The various voltage-gated potassium channels share an underlying structural similarity. The α subunit is a protein molecule with 6 membrane-spanning α-helical regions, 1 to 6 (Fig. 15–1B). The pore domain is located between the regions five and six of the α subunit. Region 4 is the voltage sensor region.53,64 Apamin, found in apitoxin obtained from bee venom, is a potent blocker of a specific subset of Ca2+-activated K+ channels conferring atrial antidysrhythmic effects. The scorpion toxins scyllatoxin and tamapin block similar K+ channels although they are structurally dissimilar.57 There are more than 70 genes encoding K+ channels in the human genome.3 Human Ether- à-go-go Related Gene (hERG) encodes the α subunit that assembles with β subunit proteins to form the IKr potassium channel. The C-terminus region of the α subunit encoded by hERG has a cyclic nucleotide binding domain and an N-terminus region similar to domains involved in signal transduction in cells.64
Many xenobiotics interact with the hERG-encoded subunit of the potassium channel to reduce the current through the IKr channel and prolong the action potential duration. The hERG α subunit of the channel is particularly susceptible to xenobiotic-induced interactions because of 2 important differences from the other channels. First, region 6 of the hERG channel has aromatic side chains on the inner cavity pore that can bind to aromatic xenobiotics, for example, cisapride, haloperidol, and terfenadine.55 Additionally, the inner cavity and entrance of the hERG channel is larger than the other voltage-gated potassium channels. This larger pore can accommodate larger xenobiotics that get trapped within the pore when the channel closes.52,53,64
Calcium channel conductivity across the myocardial cell membrane is critical for the maintenance of the appropriate duration of cell membrane depolarization and for initiation of cellular contraction. The best characterized of the calcium channels are the slow (L-type), the fast (T-type), and the ryanodine receptor calcium channel. They are more prominently involved in cardiac contractility and discussed in Chapter 16.
An understanding of the basic electrophysiology of the myocardial cell is essential to understand the toxicity of xenobiotics and to plan appropriate therapy. Figure 15–2A shows the typical action potential of myocardial cell depolarization, the electrolyte fluxes responsible for the action potential, and the resulting ECG complex (Fig. 15–2B). The action potentials of the contractile and the conductive cells are also shown.
The action potential is divided into 5 phases: phase 0, depolarization; phase 1, overshoot; phase 2, plateau; phase 3, repolarization; and phase 4, resting. Phase 0 begins when the cell is excited either by a stimulus from an adjoining cell or by spontaneous depolarization of a pacemaker cell. The stimulus causes selective voltage-gated fast sodium channels (INa+) to open, resulting in rapid depolarization of the membrane. At the end of phase 0, the voltage-sensitive sodium channels close and a transient outward potassium current (ITo) occurs, resulting in a partial repolarization of the membrane—this constitutes phase 1.
During phase 2 (plateau phase), the inward depolarizing calcium currents are largely balanced by the outward repolarizing potassium currents. Voltage-sensitive calcium channels that open allow Ca2+ movement down the 5,000–10,000-fold concentration gradient into the cell. These channels are categorized based on their conductance (fast or slow) and their sensitivity to voltage changes.57 The calcium currents (mostly the “long-lasting” current) gradually decrease as the channels inactivate. Simultaneously, the outward potassium “delayed rectifier” currents, particularly the IKs (slowly activating) current increases, terminating the plateau phase of the action potential and initiating cellular repolarization (phase 3). Other, smaller, outward potassium currents (not shown in Fig. 15–2A) play a lesser role in the duration of the action potential and development of phase 3, including IKur (ultrarapid), IKr (rapidly activating), IK-Ach (acetylcholine-dependent), and IK-ATP (adenosine triphosphate–dependent) currents.
In phase 3, the rapid repolarization phase, the cell membrane repolarizes as a result of the voltage-gated currents, the delayed rectifier currents (IKr IKs), and to a lesser extent the other K+ currents, the Na+,Ca2+ exchanger current, and the sodium/potassium pump (active transport via Na+,K+-ATPase.
Phase 4 is the resting state for much of the myocardium, except the pacemaker cells, and it corresponds to diastole in the cardiac cycle. During phases 3 and 4, active transport of Na+, K+, and Ca2+ against their electrochemical gradients returns the myocyte to its baseline resting state. The transmembrane electrochemical gradient is maintained during the resting state by a Ca2+,Na+ exchange mechanism and by ATP-dependent pumps in the membrane that together move Ca2+ out of the cells.57
In the pacemaker cells during phase 4 of the action potential, gradual electrical depolarization of the membrane occurs due to potassium currents called the Ikf for “funny” or the IKh for “hyperpolarization-activated” current.6,16 The membrane potential gradually increases in these pacemaker cells until the threshold potential is reached, the fast inward sodium channels open, and the INa current initiates the next phase 0. This electrical impulse is then propagated via the His-Purkinje conducting system of the heart.
In addition to its role in myocardial contractility, Ca2+ influx is also important in pacemaker function. Although spontaneous pacemaker cell depolarization has traditionally been ascribed to inward cation current through “pacemaker channels,”16 more recent research suggests that it is actually driven by rhythmic release of calcium from the sarcoplasmic reticulum.36,37 Regardless, Ca2+ flux plays an important role in the spontaneous depolarization (phase 4) of the action potential in the sinoatrial (SA) node.38 The rate of pacemaker cell depolarization is enhanced by β-adrenergic stimulation through phosphorylation of proteins on the sarcoplasmic reticulum and by a phosphorylation-independent action of cAMP at the pacemaker channels.42 Depolarization of cells in the SA node spreads to surrounding atrial cells where it triggers the opening of fast sodium channels and causes impulse propagation. Calcium flux also allows normal propagation of electrical impulses via the specialized myocardial conduction tissues in the atrioventricular (AV) node.
During phases 0 to 2, the cell cannot be depolarized again with another stimulus; the cell is absolutely refractory. During the latter half of phase 3, as the calcium channels recover from their inactivated to their resting state, an electrical stimulus of sufficient magnitude will cause another depolarization; the cell is said to be relatively refractory. During phase 4, the cell is no longer refractory and any appropriate stimulus that reaches the threshold level will cause depolarization.
The ECG measures the sum of all electrical activity in the heart. It is used extensively in medicine and its interpretation is widely understood by physicians of nearly all disciplines. It is an invaluable diagnostic tool for patients with cardiovascular complaints. However, it is also a valuable source of information in poisoned patients and has the potential to enhance and direct their care. Although it seems obvious that an ECG would be required following exposure to a medication used for cardiovascular indications, many medications with no overt cardiovascular effects at therapeutic doses are cardiotoxic in overdose, for example loperamide. Furthermore, in patients with unknown exposures, the ECG can suggest specific xenobiotics or demonstrate electrolyte abnormalities, long before blood is drawn. For example, oropharyngeal or dermal burns in a patient whose ECG has evidence of hyperkalemia or hypocalcemia suggests exposure to hydrofluoric acid (Chap. 104).69 Alternatively, a patient manifesting signs of the opioid toxidrome with runs of torsade de pointes might have ingested methadone (Chap. 36).21 QT interval prolongation is a clue to the etiology of an overdose with an atypical antipsychotic such as quetiapine (Chap. 67). The ECG is used to predict complications of poisoning, such as seizures following a cyclic antidepressant overdose (Chap. 68). Therefore, an ECG should be examined early in the initial evaluation of most poisoned patients.
In the 1900s, Willem Einthoven graphically displayed the electrical activity of the heart and named the different waves—P, QRS, and T. He called this tracing an “elektrokardiogramme,” and was awarded a Nobel Prize in 1924 for his efforts. The acronym EKG, still employed by some authors, was derived from Einthoven’s spelling. The acronym ECG, which is consistent with our current spelling of electrocardiogram, is used throughout this text.
Since this initial description, both the normal electrophysiology of the heart and the pharmacologic effects of various xenobiotics on the ECG have been described. Despite the large number, diversity, and complexity of the various cardiac toxins, there are a limited number of electrocardiographic manifestations.
Simplistically, a positive or upward deflection on the oscilloscope is generated when an electrical force moves toward an electrical sensor or electrode, and a downward deflection occurs if the force moves away. An ECG represents the sum of movement of all electrical forces in the heart in relation to the surface electrode, and the height above baseline represents the magnitude of the force (Fig. 15–2B). Only during depolarization or repolarization does the ECG tracing leave the isoelectric baseline, because it is only during these periods that measurable net currents are flowing in the heart. During the other periods, mechanical effects are occurring in the myocardium, but large amounts of current are not flowing.
Although the reading from a single ECG lead can provide valuable information, to visualize the heart in a nearly 3-dimensional perspective, multiple leads must be assessed simultaneously. Given the cylindrical nature of both the heart and thorax, at any given moment some of these leads will record positive voltage and others negative. The lead placement that was described and refined by Einthoven forms the basis for the bipolar or limb leads, described as I, II, and III (Fig. 15–3). The Einthoven triangle is an equilateral triangle formed by the sum of these leads. Unipolar limb leads and precordial leads were subsequently added to the standard ECG. Unipolar leads were created when the limb leads were connected to a common point where the sum of the potentials from leads I, II, and III was zero. The currently used unipolar augmented (a) leads (aVR, aVL, and aVF) are based on these unipolar leads (Fig. 15–4). The voltage potential of these unipolar, “augmented leads” is small; thus, it is amplified by incorporating the voltage change of the other 2 augmented leads. Together, leads I, II, III, aVR, aVL, and aVF form the hexaxial reference system that is used to calculate the electrical axis of the heart in the frontal plane. The precordial leads, called V1 through V6, are also unipolar measurements of the change in electric potential measured from a central point to the sixth anterior and left lateral chest positions (Fig. 15–5). If V2 is placed over the right ventricle, part of the initial positive ventricular deflection (QRS complex) reflects right ventricular activation, with electrical forces moving toward the electrode. The majority of the subsequent terminal negative deflection reflects activation of other muscle tissue (septum, left ventricular wall) when the electrical forces are moving away from the electrode. Recordings from each of these 12 leads (I, II, III, aVR, aVL, aVF, V1–6) evaluate the heart from 2 different planes in 12 different positions, yielding a 3-dimensional electrical “picture” of the heart, with respect aVR, aVL, aVF, to time and voltage.
FIGURE 15–3.
The hexaxial reference system derived from the Einthoven equilateral triangle defining the electrical potential vectors of electrocardiography. The relationships of the original 3 limb leads are illustrated. The equiangular (60°) Einthoven triangle formed by leads I, II, and III is shown (dotted lines), with positive and negative poles of each of the leads indicated. Leads I, II, and III are also presented as a triaxial reference system that intersects in the center of the ventricles.

A continuous cardiac monitor often relies on recordings from one of 2 bipolar leads: a modified left chest lead (MCL1) or a lead II. The recording from an MCL1, in which the positive electrode is in the V1 position, is similar in appearance to a V1 recording on a 12-lead ECG. This lead visualizes ventricular activity well; however, lead II shows atrial activity (ie, the P wave) much more clearly. Right ventricular precordial leads (V1, V3-6R) are sensitive and specific for determining the presence of right ventricular myocardial infarctions, although specific applications to poisoning are not reported.
The ECG tracing has specific nomenclature to define the characteristic patterns. Waves refer to positive or negative deflections from baseline, such as the P, T, or U waves. A segment is defined as the distance between 2 waves, such as the ST segment, and an interval measures the duration of a wave plus a segment, such as QT or PR interval. Complexes are a group of waves without intervals or segments between them (QRS complex). Electrophysiologically, the P wave and PR interval on the ECG tracing represent the depolarization of the atria. The QRS complex represents the depolarization of the ventricles. The plateau is depicted by the ST segment and repolarization is visualized as the T wave and the QT interval (QT). The U wave, when present, generally represents an afterdepolarization (Fig. 15–6).
The P wave is the initial deflection on the ECG that occurs with the initiation of each new cardiac cycle.
The early, middle, and late portions of the P wave are represented sequentially by the electrical potential initiated by the sinus node. The impulse is propagated directly through the right atrial muscle, producing contraction. The impulse is also propagated by specialized conduction tissue across the interatrial septum, to produce contraction of the left atrium. Additionally, internodal pathways rapidly conduct the impulse to the AV node. The electrical excitation of the sinus node differs from that of the ventricular myocardium in that current is mediated primarily by Ca2+ influx via slow T-type calcium channels, following hyperpolarization by the Ikf, mixed sodium-potassium current (f for “funny”). The “funny” current is also called the IKh (h for “hyperpolarization-activated”). The vagus nerve and b-adrenergic stimulation also affect conduction across nodal tissues.6
Clinically, abnormalities of the P wave occur with xenobiotics that depress automaticity of the sinus node, causing sinus arrest and nodal or ventricular escape rhythms (β-adrenergic antagonists, calcium channel blockers). The P wave is absent in rhythms with sinus arrest, such as occurs with xenobiotics that increase vagal tone such as cardioactive steroids and cholinergics. A notched P wave suggests delayed conduction across the atrial septum and is characteristic of quinidine poisoning or atrial enlargement (Fig. 15–7). P waves decrease in amplitude as hyperkalemia becomes more severe until they become indistinguishable from the baseline (Chap. 12).
The PR interval is measured from the beginning of the P wave to the beginning of the QRS complex (normal is 120–200 milliseconds {ms}).
Despite rapid conduction by specialized conduction tissue from the SA to the AV node, the AV node delays transmission of the impulse into the ventricles, ostensibly to allow for complete atrial emptying. Thus, the PR interval represents the interval between the onset of atrial depolarization and the onset of ventricular depolarization. Children usually have more rapid conduction and a shortened PR interval, and older adults generally have a prolonged PR interval. The segment between the end of the P wave and the beginning of the QRS complex (the PQ segment) reflects atrial contraction and is usually isoelectric. Atrial repolarization coincides with the Q wave, but the ECG evidence, or atrial T waves, are obscured by the QRS complex.
Xenobiotics that decrease interatrial or AV nodal conduction cause marked prolongation of the PR segment until such conduction completely ceases. At this point, the P wave no longer relates to the QRS complex; this is AV dissociation, or complete heart block. Some xenobiotics suppress AV nodal conduction by blocking calcium channels in nodal cells, as does magnesium, β-adrenergic antagonists, or muscarinics through enhanced vagal tone. Although the therapeutic concentrations of digoxin, as well as early cardioactive steroid poisoning, cause PR prolongation through vagal tone, direct electrophysiologic effects account for the bradycardia of poisoning (Chap. 62 and Antidotes in Depth: A22).
The QRS complex is the second and typically largest deflection on the ECG. The normal QRS duration in adults varies between 60 and 100 ms. A QRS duration that is prolonged from the patient’s baseline in the setting of poisoning indicates toxicity even if the QRS is within the normal range. The normal QRS complex axis in the frontal plane lies between –30° and 90°, although most individuals have an axis between 30° and 75°. This axis will vary with the weight and age of the patient. Alterations in myocardial function will also alter the electrical axis of the heart.
The QRS complex reflects the electrical forces generated by ventricular depolarization mediated primarily by Na+ influx into the myocardial cells. Although under normal conditions both ventricles depolarize nearly simultaneously, the greater mass of the left ventricle causes it to contribute the majority of the electrical forces. The QRS complex is primarily positive in leads I and aVL on the surface ECG recording because under normal conditions the depolarization vector is directed at 60° and is thus moving toward these leads.
The simultaneous and rapid depolarization of the ventricles results in a very short period of electrical activity recorded on the electrocardiogram. Mechanical systole lasts well past the end of the QRS complex and is maintained by continued depolarization during the plateau phase of the action potential. The return to, and maintenance of, the baseline, or isoelectric potential, simply represents that the entire heart is depolarized and there is no significant net flow of current during this period.
The axis of the terminal 40 ms (0.04 seconds) of the QRS complex represents the late stages of ventricular depolarization and generally follows the direction of the overall axis. This axis is determined by examining the last small box (0.04 seconds or 40 ms) of the QRS complex on the ECG. A leftward shift of the terminal 40 ms axis of the QRS complex correlates with toxicity from xenobiotics with Na+-channel–blocking effects (Chap. 68).
In the presence of a bundle-branch block, the 2 ventricles depolarize sequentially rather than concurrently. Although, conceptually, conduction through either the left or right bundle may be affected, many xenobiotics preferentially affect the right bundle (Fig. 15–8). The specific reasons for this effect are unclear, but it is likely related to differing refractory periods of the tissues. This effect typically results in the left ventricle depolarizing slightly more rapidly than the right. The consequence on the ECG is both a widening of the QRS complex and the appearance of the right ventricular electrical forces that were previously obscured by those of the left ventricle. These changes are typically the result of the effects of a xenobiotic that blocks fast Na+ channels. Implicated xenobiotics include amantadine,56 buproprion,22 carbamazepine,30 cocaine,1 cyclic antidepressants,11,12 diphenhydramine,58 lamotrigine,45 phenothiazines, quinidine and other type IA and IC antidysrhythmics,17 and topiramate. In the setting of cyclic antidepressant poisoning, a prolonged QRS duration has both prognostic and therapeutic value (Chap. 68).8,12,25 In one prospective analysis of ECGs in cyclic antidepressant poisoned patients, the maximal limb lead QRS duration was prognostic of seizures (0% if <100 ms; 30% if greater) and ventricular dysrhythmias (0% if <160 ms; 50% if greater).12
FIGURE 15–8.
A 35-year-old woman was found unresponsive in a hallway with an empty bottle of doxepin. Note the progression from (A) a wide QRS interval (108 ms, axis +10); to (B) at >35 min: a right bundle branch block (RBBB) with an axis of –50 and a rightward shift of the terminal 40 ms of the QRS axis; and (C) in the next hour, marked improvement occurred after infusion of hypertonic sodium bicarbonate.



The terminal 40-ms axis of the QRS complex also contains information regarding the likelihood, but not the extent, of poisoning by Na+ channel blockers. In a patient poisoned by a Na+ channel blocker, the terminal portion of the QRS has a rightward deviation greater than 120°. The common abnormalities include an R wave (positive deflection) in lead aVR and an S wave (negative deflection) in leads I and aVL.33 The combination of a rightward axis shift in the terminal 40 ms of the QRS complex (Fig. 15–9) with a prolonged QT interval and a sinus tachycardia is highly specific and sensitive for cyclic antidepressant poisoning.44 Another study suggests that although ECG changes, like a prolonged QRS duration, are better at predicting severe outcomes than the cyclic antidepressant concentration, neither is very accurate.8 One retrospective study suggests that an absolute height of the terminal portion of aVR that is greater than 3 mm predicted seizures or dysrhythmias in cyclic antidepressant–poisoned patients.32 However, in infants younger than 6 months, a rightward deviation of the terminal 40-ms QRS axis is physiologic and not predictive of cyclic antidepressant toxicity.11
FIGURE 15–9.
ECG of a patient with a tricyclic antidepressant overdose. The arrows highlight prominent S wave in leads I and aVL, and the R wave in aVR demonstrates the terminal 40-ms rightward axis shift. (Used with permission from the Fellowship in Medical Toxicology, New York University School of Medicine, New York City Poison Center.)

An apparent increase in QRS complex duration and morphology, which is an elevation or distortion of the J point called a J wave or an Osborn wave (Fig. 29–3) is a common finding in patients with hypothermia.47,65 Hypermagnesemia is also associated with prolongation of the QRS complex duration. A slight narrowing of the QRS complex may occur with hypomagnesemia. Significant hyperkalemia also causes prolongation and distortion of the QRS complex.
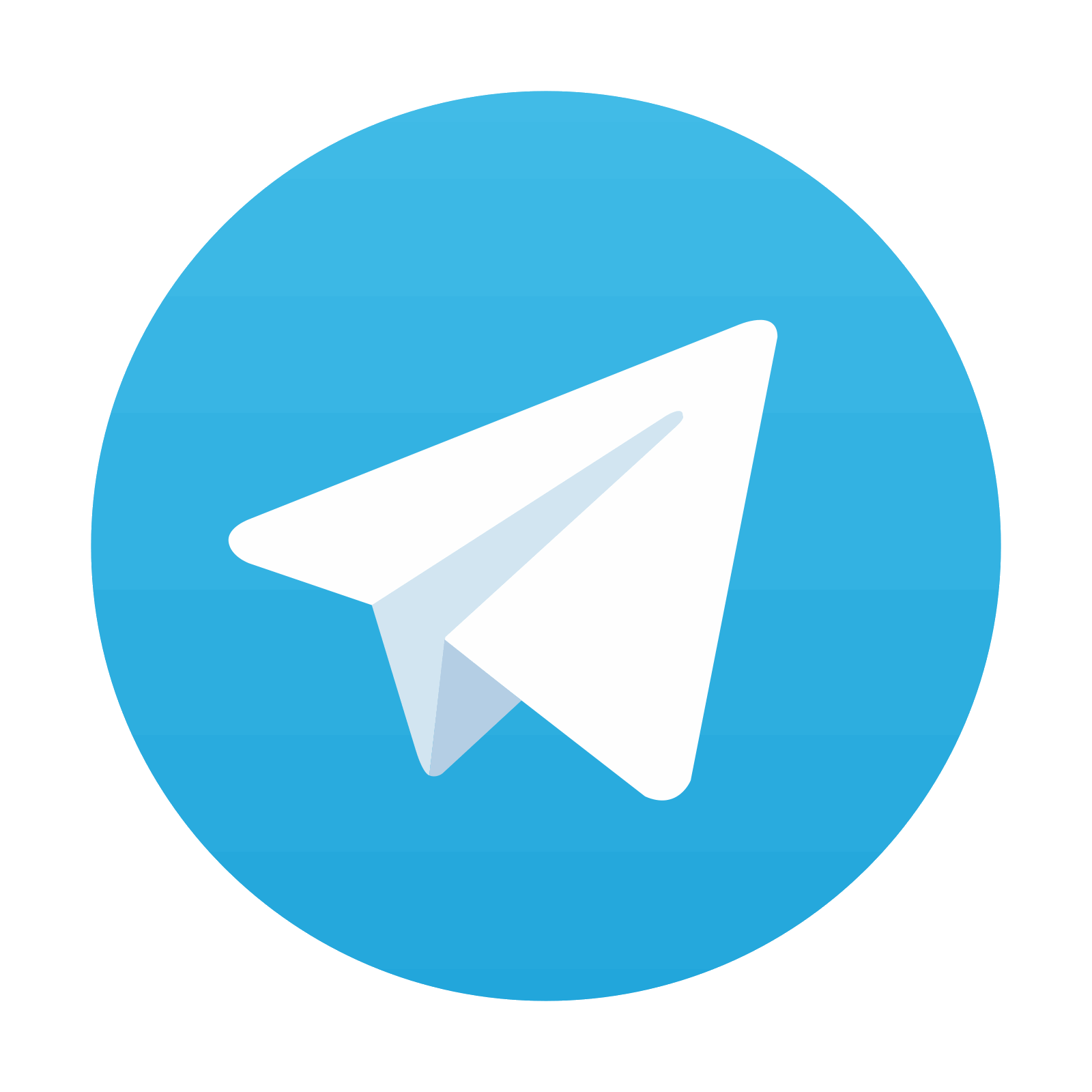
Stay updated, free articles. Join our Telegram channel
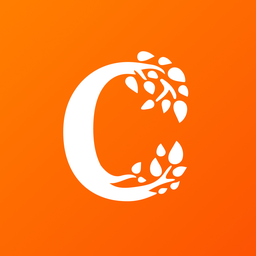
Full access? Get Clinical Tree
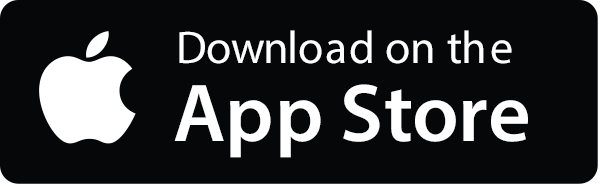
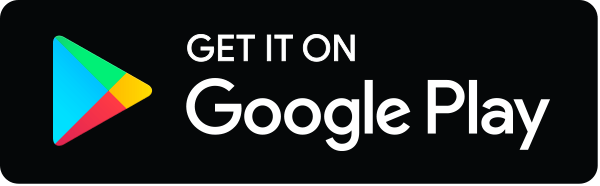