Key Concepts
The strong ion difference, Pco2, and total weak acid concentration best explain acid–base balance in physiological systems.
The bicarbonate buffer is effective against metabolic but not respiratory acid–base disturbances.
In contrast to the bicarbonate buffer, hemoglobin is capable of buffering both carbonic (CO2) and noncarbonic (nonvolatile) acids.
As a general rule, Paco2 can be expected to increase 0.25–1 mm Hg for each 1 mEq/L increase in [HCO3−].
The renal response to acidemia is 3-fold: (1) increased reabsorption of the filtered [HCO3−], (2) increased excretion of titratable acids, and (3) increased production of ammonia.
During chronic respiratory acidosis, plasma [HCO3−] increases approximately 4 mEq/L for each 10 mm Hg increase in Paco2 above 40 mm Hg.
Diarrhea is a common cause of hyperchloremic metabolic acidosis.
The distinction between acute and chronic respiratory alkalosis is not always made, because the compensatory response to chronic respiratory alkalosis is quite variable: plasma [HCO3−] decreases 2–5 mEq/L for each 10 mm Hg decrease in Paco2 below 40 mm Hg.
Vomiting or continuous loss of gastric fluid by gastric drainage (nasogastric suctioning) can result in marked metabolic alkalosis, extracellular volume depletion, and hypokalemia.
The combination of alkalemia and hypokalemia can precipitate severe atrial and ventricular arrhythmias.
Changes in temperature affect Pco2, Po2, and pH. Both Pco2 and Po2 decrease during hypothermia, but pH increases because temperature does not appreciably alter [HCO3−]: Paco2 decreases, but [HCO3−] is unchanged.
Acid–Base Management: Introduction
Nearly all biochemical reactions in the body are dependent on maintenance of a physiological hydrogen ion concentration. The latter is tightly regulated because alterations in hydrogen ion concentration are associated with widespread organ dysfunction. This regulation—often referred to as acid–base balance—is of prime importance to anesthesiologists. Changes in ventilation and perfusion and the infusion of electrolyte-containing solutions are common during anesthesia and can rapidly alter acid–base balance.
Our understanding of acid–base balance is evolving. In the past, we focused on the concentration of hydrogen ions [H+], CO2 balance, and the base excess/deficit. We now understand that the strong ion difference (SID), Pco2, and total weak acid concentration (ATOT) best explain acid–base balance in physiological systems.
Definitions
In any aqueous solution, water molecules reversibly dissociate into hydrogen and hydroxide ions:
This process is described by the dissociation constant, KW:
The concentration of water is omitted from the denominator of this expression because it does not vary appreciably and is already included in the constant. Therefore, given [H+] or [OH−], the concentration of the other ion can be readily calculated.
Example: If [H+] = 10-8 nEq/L, then [OH−] = 10-14 ÷ 10-8 = 10-6 nEq/L.
Arterial [H+] is normally 40 nEq/L, or 40 × 10-9 mol/L. Hydrogen ion concentration is more commonly expressed as pH, which is defined as the negative logarithm (base 10) of [H+] (Figure 50–1). Normal arterial pH is therefore –log (40 × 10-9) = 7.40. Hydrogen ion concentrations between 16 and 160 nEq/L (pH 6.8–7.8) are compatible with life.
Like most dissociation constants, KW is affected by changes in temperature. Thus, the electroneutrality point for water occurs at a pH of 7.0 at 25°C, but at about a pH of 6.8 at 37°C; temperature-related changes may be important during hypothermia (see Chapter 22).
Because physiological fluids are complex aqueous solutions, other factors that affect the dissociation of water into H+ and OH− are the SID, the Pco2, and ATOT.
An acid is usually defined as a chemical species that can act as a proton (H+) donor, whereas a base is a species that can act as a proton acceptor (Brönsted–Lowry definitions). In physiological solutions, it is probably better to use Arrhenius’ definitions: An acid is a compound that contains hydrogen and reacts with water to form hydrogen ions. A base is a compound that produces hydroxide ions in water. Using these definitions, the SID becomes important, as other ions in solutions (cations and anions) will affect the dissociation constant for water, and, therefore, the hydrogen ion concentration. A strong acid is a substance that readily and almost irreversibly gives up an H+ and increases [H+], whereas a strong base avidly binds H+ and decreases [H+]. In contrast, weak acids reversibly donate H+, whereas weak bases reversibly bind H+; both weak acids and bases tend to have less of an effect on [H+] (for a given concentration of the parent compound) than do strong acids and bases. Biological compounds are either weak acids or weak bases.
For a solution containing the weak acid HA, where
a dissociation constant, K, can be defined as follows:
The negative logarithmic form of the latter equation is called the Henderson–Hasselbalch equation:
From this equation, it is apparent that the pH of this solution is related to the ratio of the dissociated anion to the undissociated acid.
The problem with this approach is that it is phenomenological—measure the pH and bicarbonate, and then other variables can be manipulated mathematically. This approach works well with pure water—the concentration of [H+] must equal [OH−]. But physiological solutions are far more complex. Even in such a complex solution, the [H+] can be predicted using three variables: the SID, the Pco2, and ATOT.
The SID is the sum of all the strong, completely or almost completely dissociated, cations (Na+, K+, Ca2+, Mg2+) minus the strong anions (Cl−, lactate−, etc.) (Figure 50–2). Although we can calculate the SID, because the laws of electroneutrality must be observed, if there is a SID, other unmeasured ions must be present. Pco2 is an independent variable, assuming ventilation is ongoing. The conjugate base of HA is A− and is composed mostly of phosphates and proteins that do not change independent of the other two variables. A− plus AH is an independent variable because its value is not determined by any other variable. Note that [H+] is not a strong ion (water does not completely dissociate), but it can, does, and must change in response to any change in SID, Pco2, or ATOT to comply with the laws of electroneutrality and conservation of mass. Strong ions cannot be made to achieve electroneutrality, but hydrogen ions, H+, are created or consumed based on changes in the dissociation of water.
Figure 50–2
The strong ion difference (SID). SIDa, apparent strong ion difference. SIDe, effective strong ion difference. The strong ion gap (SIG) is the difference between SIDa and SIDe and represents the anion gap. (Reproduced, with permission, from Greenbaum J, Nirmalan M: Acid-base balance: Stewart’s physiochemical approach, Curr Anaesth Crit Care June 2005;16(3):133-135.)
As discussed above, when the weak acid HA is in solution, HA can act as an acid by donating an H+, and A− can act as a base by taking up H+. A− is therefore often referred to as the conjugate base of HA. A similar concept can be applied for weak bases. Consider the weak base B, where
BH+ is therefore the conjugate acid of B.
A buffer is a solution that contains a weak acid and its conjugate base or a weak base and its conjugate acid (conjugate pairs). Buffers minimize any change in [H+] by readily accepting or giving up hydrogen ions. It is readily apparent that buffers are most efficient in minimizing changes in the [H+] of a solution (ie, [A−] = [HA]) when pH = pK. Moreover, the conjugate pair must be present in significant quantities in solution to act as an effective buffer.
A clear understanding of acid–base disorders and compensatory physiological responses requires precise terminology (Table 50–1). The suffix “-osis” is used here to denote any pathological process that alters arterial pH. Thus, any disorder that tends to reduce pH to a less than normal value is an acidosis, whereas one tending to increase pH is termed an alkalosis. If the disorder primarily affects [HCO3−], it is termed metabolic. If the disorder primarily affects Paco2, it is termed respiratory. Secondary compensatory responses (see below) should be referred to as just that and not as an “-osis.” One might therefore refer to a metabolic acidosis with respiratory compensation.
When only one pathological process occurs by itself, the acid–base disorder is considered to be simple. The presence of two or more primary processes indicates a mixed acid–base disorder.
The suffix “-emia” is used to denote the net effect of all primary processes and compensatory physiological responses (see below) on arterial blood pH. Because arterial blood pH is normally 7.35–7.45 in adults, the term acidemia signifies a pH <7.35, whereas alkalemia signifies a pH >7.45.
Compensatory Mechanisms
Physiological responses to changes in [H+] are characterized by three phases: (1) immediate chemical buffering, (2) respiratory compensation (whenever possible), and (3) a slower but more effective renal compensatory response that may nearly normalize arterial pH even if the pathological process remains present.
Physiologically important buffers in humans include bicarbonate (H2CO3/HCO3−), hemoglobin (HbH/Hb−), other intracellular proteins (PrH/Pr−), phosphates (H2PO4−/HPO42−), and ammonia (NH3/NH4+). The effectiveness of these buffers in the various fluid compartments is related to their concentration. Bicarbonate is the most important buffer in the extracellular fluid compartment. Hemoglobin, though restricted inside red blood cells, also functions as an important buffer in blood. Other proteins probably play a major role in buffering the intracellular fluid compartment. Phosphate and ammonium ions are important urinary buffers.
Buffering of the extracellular compartment can also be accomplished by the exchange of extracellular H+ for Na+ and Ca2+ ions from bone and by the exchange of extracellular H+ for intracellular K+. Acid loads can demineralize bone and release alkaline compounds (CaCO3 and CaHPO4). Alkaline loads (NaHCO3) increase the deposition of carbonate in bone.
Buffering by plasma bicarbonate is almost immediate, whereas that due to interstitial bicarbonate requires 15–20 min. In contrast, buffering by intracellular proteins and bone is slower (2–4 h). Up to 50% to 60% of acid loads may ultimately be buffered by bone and intracellular buffers.
Although in the strictest sense, the bicarbonate buffer consists of H2CO3 and HCO3−, CO2 tension (Pco2) may be substituted for H2CO3 because:
This hydration of CO2 is catalyzed by carbonic anhydrase. If adjustments are made in the dissociation constant for the bicarbonate buffer and if the solubility coefficient for CO2 (0.03 mEq/L) is taken into consideration, the Henderson–Hasselbalch equation for bicarbonate can be written as follows:
where pK′ = 6.1.
Note that its pK′ is well removed from the normal arterial pH of 7.40, which means that bicarbonate would not be expected to be an efficient extracellular buffer (see above). The bicarbonate system is, however, important for two reasons: (1) bicarbonate (HCO3−) is present in relatively high concentrations in extracellular fluid, and (2) more importantly—Paco2 and plasma [HCO3−] are closely regulated by the lungs and the kidneys, respectively. The ability of these two organs to alter the [HCO3−]/Paco2 ratio allows them to exert important influences on arterial pH.
A simplified and more practical derivation of the Henderson–Hasselbalch equation for the bicarbonate buffer is as follows:
This equation is very useful clinically because pH can be readily converted to [H+] (Table 50–2). Note that below 7.40, [H+] increases 1.25 nEq/L for each 0.01 decrease in pH; above 7.40, [H+] decreases 0.8 nEq/L for each 0.01 increase in pH.
Example: If arterial pH = 7.28 and Paco2 = 24 mm Hg, what should the plasma [HCO3−] be?
Therefore,
It should be emphasized that the bicarbonate buffer is effective against metabolic but not respiratory acid–base disturbances. If 3 mEq/L of a strong nonvolatile acid, such as HCl, is added to extracellular fluid, the following reaction takes place:
Note that HCO3− reacts with H+ to produce CO2. Moreover, the CO2 generated is normally eliminated by the lungs such that Paco2 does not change. Consequently, [H+] = 24 × 40 ÷ 21 = 45.7 nEq/L, and pH = 7.34. Furthermore, the decrease in [HCO3−] reflects the amount of nonvolatile acid added.
In contrast, an increase in CO2 tension (volatile acid) has a minimal effect on [HCO3]. If, for example, Paco2 increases from 40 to 80 mm Hg, the dissolved CO2 increases only from 1.2 mEq/L to 2.2 mEq/L. Moreover, the equilibrium constant for the hydration of CO2 is such that an increase of this magnitude minimally drives the reaction to the left:
If the valid assumption is made that [HCO3−] does not appreciably change, then
[H+] therefore increases by 40 nEq/L, and because HCO3− is produced in a 1:1 ratio with H+, [HCO3−] also increases by 40 nEq/L. Thus, extracellular [HCO3−] increases negligibly, from 24 mEq/L to 24.000040 mEq/L. Therefore, the bicarbonate buffer is not effective against increases in Paco2, and changes in [HCO3−] do not reflect the severity of a respiratory acidosis.
Hemoglobin is rich in histidine, which is an effective buffer from pH 5.7 to 7.7 (pKa 6.8). Hemoglobin is the most important noncarbonic buffer in extracellular fluid. Simplistically, hemoglobin may be thought of as existing in red blood cells in equilibrium as a weak acid (HHb) and a potassium salt (KHb). In contrast to the bicarbonate buffer, hemoglobin is capable of buffering both carbonic (CO2) and noncarbonic (nonvolatile) acids:
Changes in alveolar ventilation responsible for the respiratory compensation of Paco2 are mediated by chemoreceptors within the brainstem (see Chapter 23). These receptors respond to changes in cerebrospinal spinal fluid pH. Minute ventilation increases 1–4 L/min for every (acute) 1 mm Hg increase in Paco2. In fact, the lungs are responsible for eliminating the approximately 15 mEq of CO2 produced every day as a byproduct of carbohydrate and fat metabolism. Respiratory compensatory responses are also important in defending against marked changes in pH during metabolic disturbances.
Decreases in arterial blood pH stimulate medullary respiratory centers. The resulting increase in alveolar ventilation lowers Paco2 and tends to restore arterial pH toward normal. The respiratory response to lower Paco2 occurs rapidly but may not reach a predictably steady state until 12–24 hr; pH is never completely restored to normal. Paco2 normally decreases 1–1.5 mm Hg below 40 mm Hg for every 1 mEq/L decrease in plasma [HCO3−].
Increases in arterial blood pH depress respiratory centers. The resulting alveolar hypoventilation tends to elevate Paco2 and restore arterial pH toward normal. The respiratory response to metabolic alkalosis is generally less predictable than the respiratory response to metabolic acidosis. Hypoxemia, as a result of progressive hypoventilation, eventually activates oxygen-sensitive chemoreceptors; the latter stimulates ventilation and limits the compensatory respiratory response. Consequently, Paco2 usually does not increase above 55 mm Hg in response to metabolic alkalosis. As a general rule, Paco2 can be expected to increase 0.25–1 mm Hg for each 1 mEq/L increase in [HCO3−].
The ability of the kidneys to control the amount of HCO3− reabsorbed from filtered tubular fluid, form new HCO3−, and eliminate H+ in the form of titratable acids and ammonium ions (see Chapter 29) allows them to exert a major influence on pH during both metabolic and respiratory acid–base disturbances. In fact, the kidneys are responsible for eliminating the approximately 1 mEq/kg per day of sulfuric acid, phosphoric acid, and incompletely oxidized organic acids that are normally produced by the metabolism of dietary and endogenous proteins, nucleoproteins, and organic phosphates (from phosphoproteins and phospholipids). Metabolism of nucleoproteins also produces uric acid. Incomplete combustion of fatty acids and glucose produces keto acids and lactic acid. Endogenous alkali are produced during the metabolism of some anionic amino acids (glutamate and aspartate) and other organic compounds (citrate, acetate, and lactate), but the quantity is insufficient to offset the endogenous acid production.
The renal response to acidemia is 3-fold: (1) increased reabsorption of the filtered HCO3−, (2) increased excretion of titratable acids, and (3) increased production of ammonia. Although these mechanisms are probably activated immediately, their effects are generally not appreciable for 12–24 hr and may not be maximal for up to 5 days.
Bicarbonate reabsorption is shown in Figure 50–3. CO2 within renal tubular cells combines with water in the presence of carbonic anhydrase. The carbonic acid (H2CO3) formed rapidly dissociates into H+ and HCO3−. Bicarbonate ion then enters the bloodstream while the H+ is secreted into the renal tubule, where it reacts with filtered HCO3− to form H2CO3. Carbonic anhydrase associated with the luminal brush border catalyzes the dissociation of H2CO3 into CO2 and H2O. The CO2
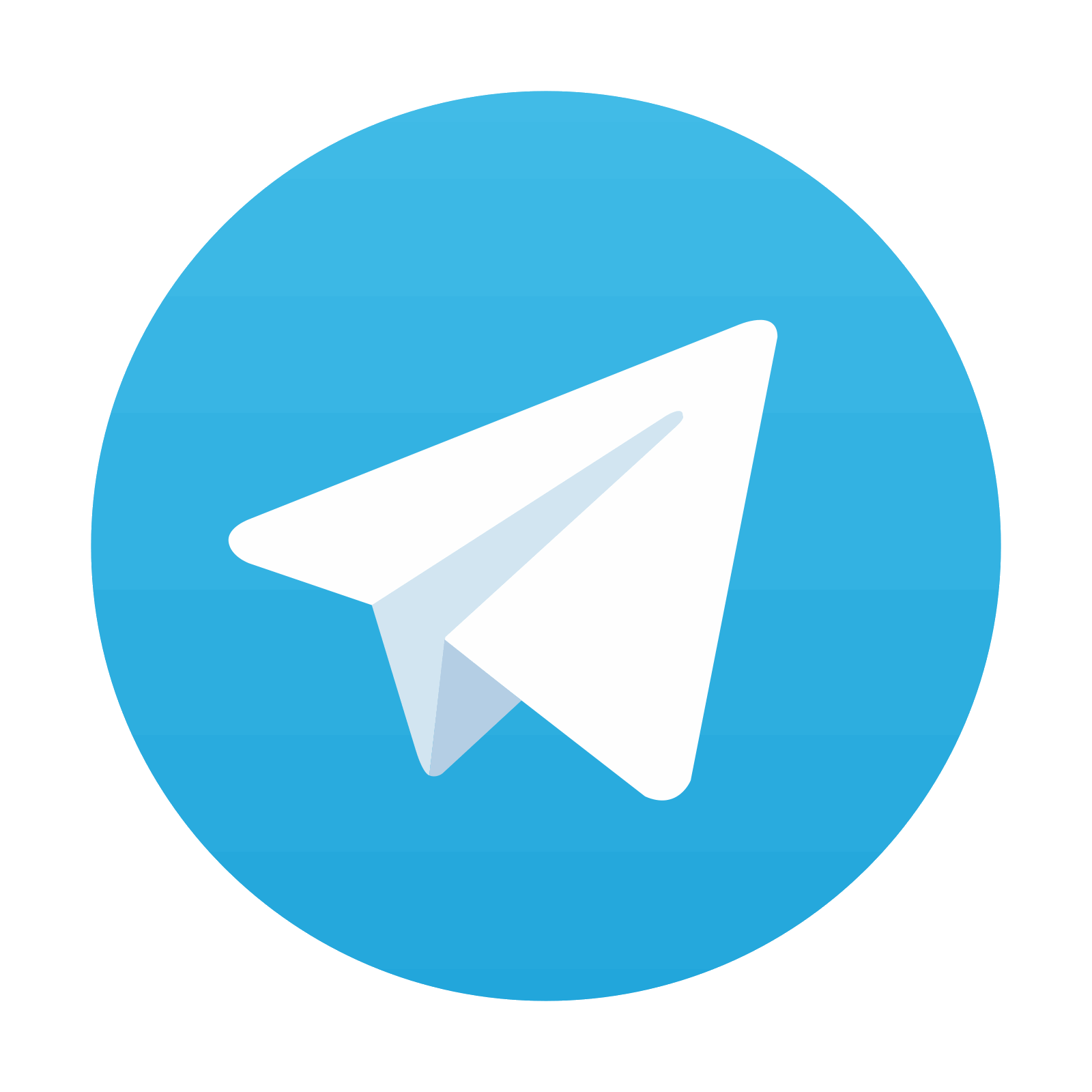
Stay updated, free articles. Join our Telegram channel
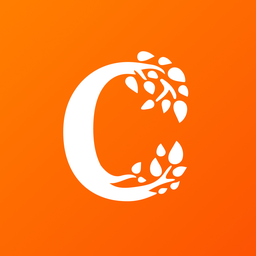
Full access? Get Clinical Tree
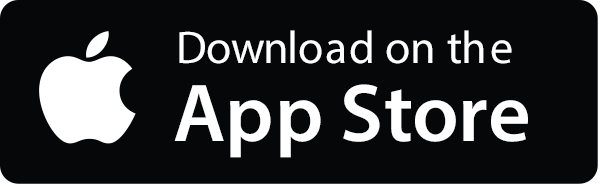
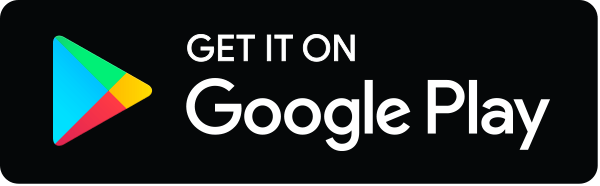
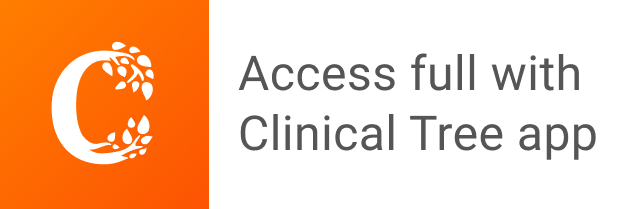