FIGURE 8.1 The basic features of a pulmonary artery (PA) catheter. Note that the PA catheter has been threaded through a large-bore introducer catheter that has a side-arm infusion port.
1. The superior vena cava pressure is identified by a venous pressure waveform, which appears as small amplitude oscillations. This pressure remains unchanged after the catheter tip is advanced into the right atrium.
2. When the catheter tip is advanced across the tricuspid valve and into the right ventricle, a pulsatile waveform appears. The peak (systolic) pressure is a function of the strength of right ventricular contraction, and the lowest (diastolic) pressure is equivalent to the right-atrial pressure.
3. When the catheter moves across the pulmonic valve and into a main pulmonary artery, the pressure waveform shows a sudden rise in diastolic pressure with no change in the systolic pressure. The rise in diastolic pressure is caused by resistance to flow in the pulmonary circulation.
4. As the catheter is advanced along the pulmonary artery, the pulsatile waveform disappears, leaving a nonpulsatile pressure that is typically at the same level as the diastolic pressure of the pulsatile waveform. This is the pulmonary artery wedge pressure, or simply the wedge pressure, and is a reflection of the filling pressure on the left side of the heart (see the next section).
5. When the wedge pressure tracing appears, the catheter is left in place (not advanced further). The balloon is then deflated, and the pulsatile pressure waveform should reappear. The catheter is then secured in place, and the balloon is left deflated.
On occasion, the pulsatile pressure in the pulmonary arteries never disappears despite advancing the catheter maximally (unexplained observation). If this occurs, the pulmonary artery diastolic pressure can be used as a surrogate measure of the wedge pressure (the two pressures should be equivalent in the absence of pulmonary hypertension).
THE WEDGE PRESSURE
The wedge pressure is obtained by slowly inflating the balloon at the tip of the PA catheter until the pulsatile pressure disappears, as shown in Figure 8.3. Note that the wedge pressure is at the same level as the diastolic pressure in the pulmonary artery. This relationship is altered in pulmonary hypertension, where the wedge pressure is lower than the pulmonary artery diastolic pressure.
FIGURE 8.2 The pressure waveforms at different points along the normal course of a pulmonary artery catheter. These waveforms are used to identify the location of the catheter tip as it is advanced.
Wedge Pressure Tracing
The wedge pressure represents the venous pressure on the left side of the heart, and the magnified section of the wedge pressure in Figure 8.3 shows a typical venous contour that is similar to the venous pressure on the right side of the heart. The a wave is produced by left atrial contraction, the c wave is produced by closure of the mitral valve (during isometric contraction of the left ventricle), and the v wave is produced by systolic contraction of the left ventricle against a closed mitral valve. These components are often difficult to distinguish, but prominent v waves are readily apparent in patients with mitral regurgitation.
Principle of the Wedge Pressure
The principle of the wedge pressure is illustrated in Figure 8.4. When the balloon on the PA catheter is inflated to obstruct flow (Q=0), there is a static column of blood between the tip of the catheter and the left atrium, and the wedge pressure at the tip of the catheter (PW) is equivalent to the pulmonary capillary pressure (Pc) and the pressure in the left atrium (PLA). To summarize: if Q=0, then PW = Pc = PLA. If the mitral valve is behaving normally, the left atrial pressure (wedge pressure) will be equivalent to the end-diastolic pressure (the filling pressure) of the left ventricle. Therefore, in the absence of mitral valve disease, the wedge pressure is a measure of left ventricular filling pressure.
FIGURE 8.3 Pressure tracing showing the transition from a pulsatile pulmonary artery pressure to a balloon occlusion (wedge) pressure. The magnified area shows the components of the wedge pressure: a wave (atrial contraction), c wave (mitral valve closure), and v wave (ventricular contraction).
Influence of Alveolar Pressure
The wedge pressure will reflect left atrial pressure only if the pulmonary capillary pressure is greater than the alveolar pressure (Pc>PA in Figure 8.4); otherwise the wedge pressure will reflect the alveolar pressure. Capillary pressure exceeds alveolar pressure when the tip of the PA catheter is below the level of the left atrium, or posterior to the left atrium in the supine position. Most PA catheters enter dependent lung regions naturally (because the blood flow is highest in these regions), and lateral chest x-rays are rarely obtained to verify catheter tip position.
FIGURE 8.4 The principle of the wedge pressure measurement. When flow ceases because of balloon inflation (Q=0), the wedge pressure (PW) is equivalent to the pulmonary capillary pressure (Pc) and the pressure in the left atrium (PLA). This occurs only in the most dependent lung region, where the pulmonary capillary pressure (Pc) is greater than the alveolar pressure (PA).
Respiratory variations in the wedge pressure suggest that the catheter tip is in a region where alveolar pressure exceeds capillary pressure (7). In this situation, the wedge pressure should be measured at the end of expiration, when the alveolar pressure is closest to atmospheric (zero) pressure. The influence of intrathoracic pressure on cardiac filling pressures is described in more detail in Chapter 9.
Spontaneous Variations
In addition to respiratory variations, the CVP and wedge pressures can vary spontaneously, independent of any change in the factors that influence these pressures. The spontaneous variation in wedge pressure is ″4 mm Hg in 60% of patients, but it can be as high as 7 mm Hg (8). In general, a change in the wedge pressure should exceed 4 mm Hg to be considered a clinically significant change.
Wedge vs. Hydrostatic Pressure
The wedge pressure is often mistaken as the hydrostatic pressure in the pulmonary capillaries, but this is not the case (9,10). The wedge pressure is measured in the absence of blood flow. When the balloon is deflated and flow resumes, the pressure in the pulmonary capillaries (Pc) will be higher than the pressure in the left atrium (PLA), and the difference in pressures will be dependent on the flow rate (Q) and the resistance to flow in the pulmonary veins (RV); i.e.,
(8.1)
Since the wedge pressure is equivalent to left atrial pressure, Equation 8.1 can be restated using the wedge pressure (PW) as a substitute for left atrial pressure (PLA).
(8.2)
Therefore the wedge pressure and capillary hydrostatic pressure must be different to create a pressure gradient for venous flow to the left side of the heart. The magnitude of this difference is unclear because it is not possible to determine RV. However, the discrepancy between wedge and capillary hydrostatic pressures may be magnified in ICU patients because conditions that promote pulmonary venoconstriction (i.e., increase RV), such as hypoxemia, endotoxemia, and the acute respiratory distress syndrome (11,12), are common in these patients.
Wedge Pressure in ARDS
The wedge pressure is used to differentiate hydrostatic pulmonary edema from the acute respiratory distress syndrome (ARDS); a normal wedge pressure is considered evidence of ARDS (13). However, since the capillary hydrostatic pressure is higher than the wedge pressure, a normal wedge pressure measurement will not rule out the diagnosis of hydrostatic pulmonary edema. Therefore, the use of a normal wedge pressure as a diagnostic criterion for ARDS should be abandoned.
THERMODILUTION CARDIAC OUTPUT
The ability to measure cardiac output increases the monitoring capacity of the PA catheter from 2 parameters (i.e., central venous pressure and wedge pressure) to at least 10 parameters (see Tables 8.1 and 8.2), and allows a physiologic evaluation of cardiac performance and systemic oxygen transport.
FIGURE 8.5 The thermodilution method of measuring cardiac output. See text for explanation.
The indicator-dilution method of measuring blood flow is based on the premise that, when an indicator substance is added to circulating blood, the rate of blood flow is inversely proportional to the change in concentration of the indicator over time. If the indicator is a temperature, the method is known as thermodilution.
The thermodilution method is illustrated in Figure 8.5. A dextrose or saline solution that is colder than blood is injected through the proximal port of the catheter in the right atrium. The cold fluid mixes with blood in the right heart chambers, and the cooled blood is ejected into the pulmonary artery and flows past the thermistor on the distal end of the catheter. The thermistor records the change in blood temperature with time; the area under this curve is inversely proportional to the flow rate in the pulmonary artery, which is equivalent to the cardiac output in the absence of intracardiac shunts. Electronic monitors integrate the area under the temperature–time curves and provide a digital display of the calculated cardiac output.
Thermodilution Curves
Examples of thermodilution curves are shown in Figure 8.6. The low cardiac output curve (upper panel) has a gradual rise and fall, whereas the high output curve (middle panel) has a rapid rise, an abbreviated peak, and a steep downslope. Note that the area under the low cardiac output curve is greater than the area under the high output curve (i.e., the area under the curves is inversely related to the flow rate).
Sources of Error
Serial measurements are recommended for each cardiac output determination. Three measurements are sufficient if they differ by 10% or less, and the cardiac output is taken as the average of all measurements. Serial measurements that differ by more than 10% are considered unreliable (14).
Variability
Thermodilution cardiac output can vary by as much as 10% without any apparent change in the clinical condition of the patient (15). Therefore, a change in thermodilution cardiac output should exceed 10% to be considered clinically significant.
Tricuspid Regurgitation
Regurgitant flow across the tricuspid valve can be common during positive-pressure mechanical ventilation. The regurgitant flow causes the indicator fluid to be recycled, producing a prolonged, low-amplitude thermodilution curve similar to the one in the bottom frame of Figure 8.6. This results in a falsely low cardiac output measurement (16).
Intracardiac Shunts
Intracardiac shunts produce falsely high thermodilution cardiac output measurements. In right-to-left shunts, a portion of the cold indicator fluid passes through the shunt, thereby creating an abbreviated thermodilution curve similar to the high-output curve in the middle panel of Figure 8.6. In left-to-right shunts, the thermodilution curve is abbreviated be-cause the shunted blood increases the blood volume in the right heart chambers, and this dilutes the indicator solution that is injected.
FIGURE 8.6 Thermodilution curves for a low cardiac output (upper panel), a high cardiac output (middle panel), and tricuspid insufficiency (lower panel). The sharp inflection in each curve marks the end of the measurement period. CO = cardiac output.
HEMODYNAMIC PARAMETERS
The PA catheter provides a wealth of information on cardiovascular function and systemic oxygen transport. This section provides a brief de-scription of the hemodynamic parameters that can be measured or de-rived with the PA catheter. These parameters are included in Table 8.1.
Body Size
Hemodynamic parameters are often expressed in relation to body size, and the popular measure of body size for hemodynamic measurements is the body surface area (BSA), which can be determined with the following simple equation (17).
(8.3)
Why not use body weight to adjust for body size? BSA was chosen for hemodynamic measurements because cardiac output is linked to metabolic rate, and the basal metabolic rate is expressed in terms of body surface area. The average-sized adult has a body surface area of 1.7 m2.
Table 8.1 Hemodynamic and Oxygen Transport Parameters
Cardiovascular Parameters
The following parameters are used to evaluate cardiac performance and mean arterial pressure. The normal ranges for these parameters are in-cluded in Table 8.1. Parameters that are adjusted for body surface area are identified by the term index.
Central Venous Pressure
When the PA catheter is properly placed, the proximal port of the catheter should be situated in the right atrium, and the pressure recorded from this port should be the right atrial pressure (RAP). As mentioned previously, the pressure in the right atrium is the same as the pressure in the superior vena cava, and these pressures are collectively called the central venous pressure (CVP). In the absence of tricuspid valve dysfunction, the CVP should be equivalent to the right-ventricular end-diastolic pressure (RVEDP).
(8.4)
The CVP is used as a measure of the right ventricular filling pressure. The normal range for the CVP is 0–5 mm Hg, and it can be a negative pressure in the sitting position. The CVP is a popular measurement in critical care, and is described in more detail in the next chapter.
Pulmonary Artery Wedge Pressure
The pulmonary artery wedge pressure (PAWP) is described earlier in the chapter. The PAWP is a measure of left-atrial pressure (LAP), which is equivalent to the left-ventricular end-diastolic pressure (LVEDP) when mitral valve function is normal.
(8.5)
The wedge pressure is a measure of the left ventricular filling pressure. It is slightly higher than the CVP (to keep the foramen ovale closed), and the normal range is 6–12 mm Hg.
Cardiac Index
The thermodilution cardiac output (CO) is the average stroke output of the heart in one-minute periods. It is typically adjusted to body surface area (BSA), and is called the cardiac index (CI).
(8.6)
In the average-sized adult, the cardiac index is about 60% of the cardiac output, and the normal range is 2.4–4 L/min/m2.
Stroke Index
The heart is a stroke pump, and the stroke volume is the volume of blood ejected in one pumping cycle. The stroke volume is equivalent to the average stroke output of the heart per minute (the measured cardiac output) divided by the heart rate (HR). When cardiac index (CI) is used, the stroke volume is called the stroke index (SI).
(8.7)
The stroke index is a measure of the systolic performance of the heart during one cardiac cycle. The normal range in adults is 20–40 mL/m2.
Systemic Vascular Resistance Index
The hydraulic resistance in the systemic circulation is not a measurable quantity for a variety of reasons (e.g., resistance is flow-dependent and varies in different regions). Instead, the systemic vascular resistance (SVR) is a global measure of the relationship between systemic pressure and flow. The SVR is directly related to the pressure drop from the aorta to the right atrium (MAP – CVP), and inversely related to the cardiac output (CI).
(8.8)
The SVRI is expressed in Wood units (mm Hg/L/min/m2), which can be multiplied by 80 to obtain more conventional units of resistance (dynes•sec–1•cm-5/m2), but this conversion offers no advantage (18).
Pulmonary Vascular Resistance Index
The pulmonary vascular resistance (PVR) has the same limitations as mentioned for the systemic vascular resistance. The PVR is a global measure of the relationship between pressure and flow in the lungs, and is derived as the pressure drop from the pulmonary artery to the left atrium, divided by the cardiac output. Because the pulmonary artery wedge pressure (PAWP) is equivalent to the left atrial pressure, the pressure gradient across the lungs can be expressed as the difference between the mean pulmonary artery pressure and the wedge pressure (PAP – PAWP).
(8.9)
Like the SVRI, the PVRI is expressed in Wood units (mm Hg/L/min/m2), which can be multiplied by 80 to obtain more conventional units of resistance (dynes•sec-1•cm–5/m2).
Oxygen Transport Parameters
The oxygen transport parameters provide a global (whole body) measure of oxygen supply and oxygen consumption. These parameters are de-scribed in detail in Chapter 10 and are presented only briefly here.
Oxygen Delivery
The rate of oxygen transport in arterial blood is called the oxygen delivery (DO2), and is the product of the cardiac output (or CI) and the oxygen concentration in arterial blood (CaO2).
(8.10)
The O2 concentration in arterial blood (CaO2) is a function of the hemoglobin concentration (Hb) and the percent saturation of hemoglobin with oxygen (SaO2): CaO2 = 1.3 × Hb × SaO2. Therefore, the DO2 equation can be rewritten as:
(8.11)
DO2 is expressed as mL/min/m2 (if the cardiac index is used instead of the cardiac output), and the normal range is shown in Table 8.1.
Oxygen Uptake
Oxygen uptake (VO2), also called oxygen consumption, is the rate at which oxygen is taken up from the systemic capillaries into the tissues. The VO2 is calculated as the product of the cardiac output (or CI) and the difference in oxygen concentration between arterial and venous blood (CaO2 – CvO2). The venous blood in this instance is “mixed” venous blood in the pulmonary artery.
(8.12)
If the CaO2 and CvO2 are each broken down into their component parts, the VO2 equation can be rewritten as:
(8.13)
(where SaO2 and SvO2 are the oxyhemoglobin saturations in arterial and mixed venous blood, respectively). VO2 is expressed as mL/min/m2 (when the cardiac index is used instead of the cardiac output), and the normal range is shown in Table 8.1. An abnormally low VO2 (<100 mL/min/m2) is evidence of impaired aerobic metabolism.
Oxygen Extraction Ratio
The oxygen extraction ratio (O2ER) is the fractional uptake of oxygen from the systemic microcirculation, and is equivalent to the ratio of O2 uptake to O2 delivery. Multiplying the ratio by 100 expresses it as a percent.
(8.14)
The O2ER is a measure of the balance between O2 delivery and O2 uptake. It is normally about 25%, which means that 25% of the oxygen delivered to the systemic capillaries is taken up into the tissues.
APPLICATIONS
Hemodynamic Patterns
Most hemodynamic problems can be identified by noting the pattern of changes in three hemodynamic parameters: cardiac filling pressure (CVP or PAWP), cardiac output, and systemic or pulmonary vascular resistance. This is demonstrated in Table 8.2 using the three classic forms of shock: hypovolemic, cardiogenic, and vasogenic. Each of these conditions produces a distinct pattern of changes in the three parameters. Since there are 3 parameters and 3 possible conditions (low, normal, or high), there are 33 or 27 possible hemodynamic patterns, each representing a distinct hemodynamic condition.
Table 8.2 Hemodynamic Patterns in Different Types of Shock
Tissue Oxygenation
The hemodynamic patterns just described can identify a hemodynamic problem, but they provide no information about the impact of the problem on tissue oxygenation. The addition of the oxygen uptake (VO2) will correct this shortcoming, and can help identify a state of clinical shock. Clinical shock can be defined as a condition where tissue oxygenation is inadequate for the needs of aerobic metabolism. Since a VO2 that is below normal can be used as indirect evidence of oxygen-limited aerobic metabolism, a subnormal VO2 can be used as indirect evidence of clinical shock. The following example shows how the VO2 can add to the evaluation of a patient with cardiac pump failure.
Table 8.3 Compensated Heart Failure vs. Cardiogenic Shock
Without the VO2 measurement in Table 8.3, it is impossible to differentiate compensated heart failure from cardiogenic shock. This illustrates how oxygen transport monitoring can be used to determine the consequences of hemodynamic abnormalities on systemic oxygenation. Oxygen transport monitoring is described in more detail in Chapter 10.
A FINAL WORD
Despite the wealth of physiologically relevant information provided by the PA catheter, the catheter has been vilified and almost abandoned in recent years because clinical studies have shown added risk with little or no survival benefit, associated with use of the catheter (2–4). The following points are made in support of the PA catheter.
1. First and foremost, the PA catheter is a monitoring device, not a therapy. If a PA catheter is placed to evaluate a problem and it uncovers a disorder that is untreatable (e.g., cardiogenic shock), the problem is not the catheter, but a lack of effective therapy. Clinical outcomes should be used to evaluate therapies, not measurements.
2. In addition, surveys indicate that physicians often don’t understand the measurements provided by PA catheters (19,20). Any tool can be a weapon in the wrong hands.
3. Finally, the incessant use of mortality rates to evaluate critical care interventions is problematic because the presumption that every intervention has to save lives to be of value is flawed. Interventions should (and do) have more specific and immediate goals other than life or death. In the case of a monitoring device, the goal is to provide clinical information, and the PA catheter achieves this goal with distinction.
REFERENCES
1. Swan HJ. The pulmonary artery catheter. Dis Mon 1991; 37:473–543.
2. The ESCAPE Investigators. Evaluation study of congestive heart failure and pulmonary artery catheterization effectiveness: the ESCAPE trial. JAMA 2005; 294:1625–1633.
3. The NHLBI Acute Respiratory Distress Syndrome (ARDS) Clinical Trials Network. Pulmonary artery versus central venous catheter to guide treatment of acute lung injury. N Engl J Med 2006; 354:2213–2224.
4. Harvey S, Young D, Brampton W, et al. Pulmonary artery catheters for adult patients in intensive care. Cochrane Database Syst Rev 2006; 3:CD003408.
5. Chatterjee K. The Swan-Ganz catheters: past, present, and future. Circulation 2009; 119:147–152.
6. Kahwash R, Leier CV, Miller L. Role of pulmonary artery catheter in diagnosis and management of heart failure. Cardiol Clin 2011; 29:281–288.
7. O’Quin R, Marini JJ. Pulmonary artery occlusion pressure: clinical physiology, measurement, and interpretation. Am Rev Respir Dis 1983; 128:319–326.
8. Nemens EJ, Woods SL. Normal fluctuations in pulmonary artery and pulmonary capillary wedge pressures in acutely ill patients. Heart Lung 1982; 11:393–398.
9. Cope DK, Grimbert F, Downey JM, et al. Pulmonary capillary pressure: a review. Crit Care Med 1992; 20:1043–1056.
10. Pinsky MR. Hemodynamic monitoring in the intensive care unit. Clin Chest Med 2003; 24:549–560.
11. Tracey WR, Hamilton JT, Craig ID, Paterson NAM. Effect of endothelial injury on the responses of isolated guinea pig pulmonary venules to reduced oxygen tension. J Appl Physiol 1989; 67:2147–2153.
12. Kloess T, Birkenhauer U, Kottler B. Pulmonary pressure–flow relationship and peripheral oxygen supply in ARDS due to bacterial sepsis. Second Vienna Shock Forum, 1989:175–180.
13. Bernard GR, Artigas A, Brigham KL, et al. The American–European Consen-sus Conference on ARDS: definitions, mechanisms, relevant outcomes, and clinical trial coordination. Am Rev Respir Crit Care Med 1994; 149:818–824.
14. Nadeau S, Noble WH. Limitations of cardiac output measurement by thermodilution. Can J Anesth 1986; 33:780–784.
15. Sasse SA, Chen PA, Berry RB, et al. Variability of cardiac output over time in medical intensive care unit patients. Chest 1994; 22:225–232.
16. Konishi T, Nakamura Y, Morii I, et al. Comparison of thermodilution and Fick methods for measurement of cardiac output in tricuspid regurgitation. Am J Cardiol 1992; 70:538–540.
17. Mattar JA. A simple calculation to estimate body surface area in adults and its correlation with the Dubois formula. Crit Care Med 1989; 846–847.
18. Bartlett RH. Critical Care Physiology. New York: Little, Brown & Co, 1996:36.
19. Iberti TJ, Fischer EP, Liebowitz AB, et al. A multicenter study of physicians’ knowledge of the pulmonary artery catheter. JAMA 1990; 264:2928–2932.
20. Gnaegi A, Feihl F, Perret C. Intensive care physicians’ insufficient knowledge of right heart catheterization at the bedside: time to act? Crit Care Med 1997; 25:213–220.
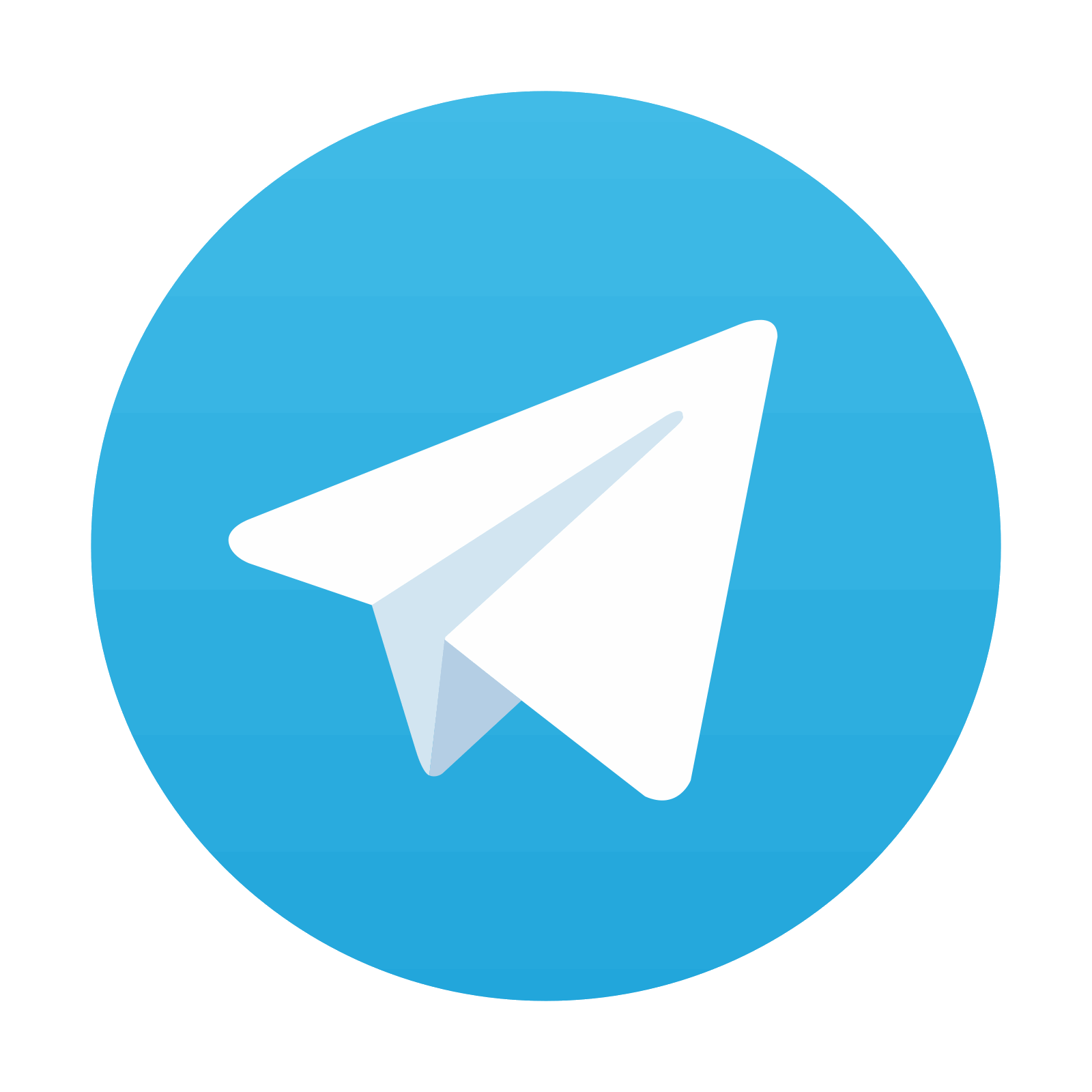
Stay updated, free articles. Join our Telegram channel
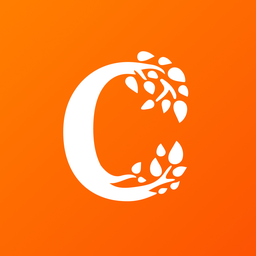
Full access? Get Clinical Tree
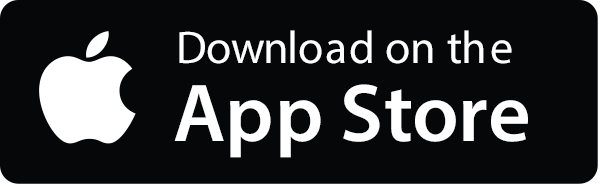
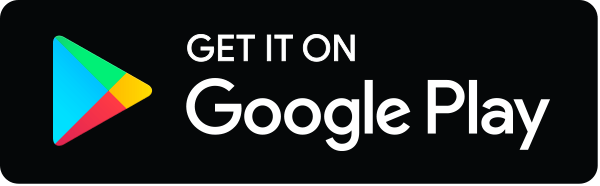