CHAPTER 5. Transport Physiology
Reneé Semonin Holleran
Competencies








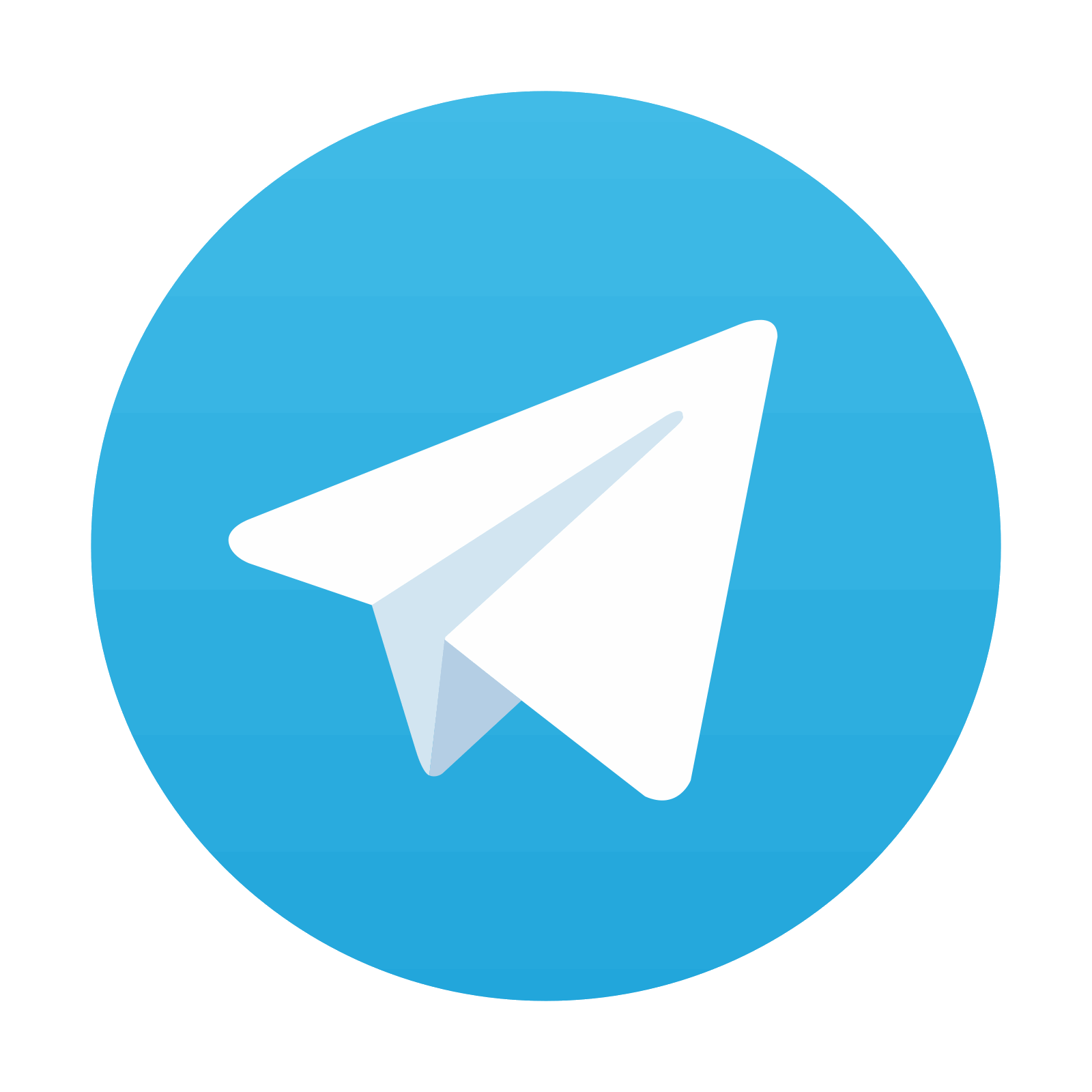
Get Clinical Tree app for offline access
1. Identify and describe the gas laws that are pertinent to the transport environment.
2. Provide appropriate interventions to prevent the adverse effects of barometric pressure changes during patient transport.
3. Identify specific management of the stresses that may occur during transport.
Patient transport requires an understanding of physiologic stresses that may occur. Understanding the concepts of transport physiology is crucial because they are the basis for the special skills used in transporting patients within the air medical environment via fixed-wing or rotor-wing aircraft.
This chapter on transport physiology includes a discussion on gas laws and their potential effect on patients and the transport team. It also includes information about the physiologic stresses of transport and effects on the patient and team during air medical transport.
THE GAS LAWS
For optimal patient care in the air medical environment, transport personnel must possess in-depth knowledge of altitude physiology and the effects on the patient during transport. Altitude physiology exemplifies the concepts of the gas laws; the primary concern is the relationships among the interdependent variables of temperature, pressure, volume, and mass of gases. Before the gas laws are addressed, those factors that influence the behavior of gases need to be considered. The four basic variables that affect gas volumetric relationships are temperature, pressure, volume, and the relative mass of a gas or the number of molecules. These variables (T, P, V, and n) are defined as follows 4,25:
1. Temperature (T), when expressed in degrees kelvin (K), indicates the level of energy of a gas sample and is referred to as absolute temperature, converted from temperature Celsius (°C) or Fahrenheit (°F).
2. Pressure (P), defined as absolute or total exerted pressure, is conventionally expressed in atmospheres (torr) or as a given column of mercury in millimeters (mm Hg) or of water balancing the pressure in centimeters (cm H 2O).
3. Volume (V) is expressed in cubic units, such as cubic meters (m 3), cubic centimeters (cm 3), or in liters (L).
4. Relative mass of a gas or number of molecules (n) or ions is expressed in gram molecules (the molecular weight of the substance in grams).
Gas laws govern the body’s physiologic response to barometric pressure changes by these four variables. When the transport team is caring for the air transport patient, these changes become particularly important on ascent and descent.
Boyle’s Law
Boyle’s law, which originated from experiments conducted by Robert Boyle in 1662, states that at a constant temperature, the volume of gas is inversely proportional to its pressure. This law applies to all gases and may be expressed as follows31:

where V 1 is initial volume, V 2 is final volume, P 1 is initial pressure, and P 2 is final pressure
Thus, at a constant temperature, the volume of a gas is inversely proportional to the pressure. The gas in a balloon, for example, expands as the balloon ascends.
Dalton’s Law (Law of Partial Pressure)
Dalton was a chemist who observed in 1803 that the total pressure of a mixture of gasses is equal to the sum of the partial pressures of each gas in the mixture. Dalton’s law is expressed in the following formula:

P is the total pressure of the gas mixture, and P 1, P 2, and P 3 are partial pressures of each gas in the mixture.
The partial pressure of each gas in the mixture is derived from the following equation31:

where P 1 is the partial pressure of gas 1, F 1 is the fractional concentration of gas 1 in the mixture, and P is the total pressure of the gas mixture. 31
In other words, gases in a mixture exert pressure equivalent to the pressure each would exert if present alone in the volume of the total mixture. Thus, each gas present in a mixture exerts a partial pressure equal to the fractional concentration (by volume) multiplied by the total pressure. 4,25
A mathematic illustration of Dalton’s law is shown in the following example, in which the partial pressure of oxygen (PO 2) at sea level is calculated:

Barometric pressure, or atmospheric pressure, is the pressure exerted against an object or a person by the atmosphere (Table 5-1). At sea level, this pressure is 15 psi. Increased altitude results in decreased barometric pressure. Barometric pressure multiplied by the concentration of a gas is equal to the partial pressure of the gas. 3,31,
Indifferent Stage | Compensatory Stage | Disturbance Stage | Critical Stage | |
---|---|---|---|---|
Altitude (thousands of feet) | 0-10 | 10-15 | 15-20 | 20-25 |
Symptoms | Decrease in night vision | Drowsiness | Physiologic responses no longer compensate for oxygen deficiency | Mental confusion |
Poor judgment | Incapacitation | |||
Impaired coordination | Seizures | |||
Mistakes in judgment | Unconsciousness | |||
Peripheral vision and central vision is impaired | Cardiovascular collapse | |||
Death | ||||
Impaired coordination | ||||
Decreased sensation of pain | ||||
Impaired judgment |

Charles’ Law
An additional development in the early formulation of the laws of ideal gases came from the French physicist Jacques Charles who concluded that, “When pressure is constant, the volume of a gas is very nearly proportional to its absolute temperature.” This law is expressed as follows31:

where V 1 is initial volume, V 2 is final volume, T 1 is initial absolute temperature, and T 2 is final absolute temperature.
Thus, the volume is directly proportional to the temperature when expressed on an absolute scale with all other factors constant (P and n are constant). 4 Consequently, if a mass of gas is kept under a constant pressure as the absolute temperature of the gas is increased or decreased, the volume increases or decreases accordingly. 7
The motion of the molecules in a gas is directly related to temperature. A decrease in the temperature of a gas causes the molecules to move more slowly, whereas an increase in the temperature causes a faster motion. The relationship shows that when a greater force is exerted, the volume expands. An example is a can of shaving cream put into a fire; one can then watch Charles’ law at work. Carrying out this experiment is not recommended.
Charles’ law describes how gas volume is affected by temperature when pressure remains constant. However, because human body temperature is generally constant, the law has a limited effect on human physiology. 31
Gay-Lussac’s Law
Gay-Lussac’s law is often combined with Charles’ law because it, too, deals with a directly proportional relationship between pressure and temperature. Gay-Lussac’s law expresses the same relationship but is stated as follows:

where V and n are constant. Thus, the pressure of a gas when volume is constant is directly proportional to the absolute temperature for a constant amount of gas. 4 For example, the pressure in an oxygen tank decreases as the temperature decreases.
Henry’s Law
Henry’s law deals with the solubility of gases in liquids. The law states: “The quantity of gas dissolved in 1 cm3 (1 mL) of a liquid is proportional to the partial pressure of the gas in contact with the liquid.” The absolute amount of any gas dissolved in liquid under conditions of equilibrium is dependent on the solubility of the gas in the liquid and the temperature, in addition to the partial pressure of the gas. 31 A simpler interpretation is that the weight of a gas dissolved in a liquid is directly proportional to the weight of the gas above the liquid. 7,39 An ideal example is a can of soda (i.e., a carbonated soft drink) that is opened immediately after it has been dropped. The soda was bottled with an equilibrium established between the soda and the gas in the can. When the can is opened, the pressure of the gas above the soda is drastically reduced, which releases the gas within the soda as bubbles. A further example of this law is decompression sickness. When a scuba diver ascends too rapidly from a deep dive, nitrogen bubbles can form in the blood and cause one form of decompression sickness.
Graham’s Law (Law of Gaseous Diffusion)
Graham’s law states that the rate of diffusion of a gas through a liquid medium is directly related to the solubility of the gas and inversely proportional to the square root of its density or gram molecular weight. 8 Gases move from a higher pressure or concentration to a region of lower pressure or concentration. b Examples are simple diffusion or gas exchange at the cellular level. 31
STRESSES OF TRANSPORT
Multiple stresses that may be caused by air medical transport have been identified. According to the US Air Force, 3,29,33 which has done the most research on the subject of stresses related to flight, the eight classic stresses of flight are as follows:
▪ Hypoxia
▪ Barometric pressure changes
▪ Thermal changes
▪ Decreased humidity
▪ Noise
▪ Vibration
▪ Fatigue
▪ Gravitational forces
Additional stresses related to transport include the following:
▪ Spatial disorientation
▪ Flicker vertigo
▪ Fuel vapors
Hypoxia
Within the air medical environment, different types of hypoxia are found. An understanding of the terms hypoxia, hypoxemia, and hypercapnia is essential to establish a foundation of knowledge about the effects of decreased partial pressure of oxygen.
Hypoxia is a general term that describes the state of oxygen deficiency in the tissues. It refers to a decrease in tissue oxygen or an oxygen supply inadequate to meet tissue needs. 1,3,16,34 Hypoxia disrupts the intracellular oxidative process and impairs cellular function. 23,34
Many factors may interfere with a blood cell’s ability to carry oxygen to the body. Anemia, altitude, alcohol, medications, carbon monoxide poisoning, and heavy smoking can all decrease the blood’s ability to absorb and transport oxygen.
Hypoxemia refers to a decrease in arterial blood oxygen tension (PaO 2). A normal PaO 2 does not guarantee adequate tissue oxygenation; conversely, a low PaO 2 may not indicate tissue hypoxia and may be clinically acceptable. 1,34
Hypercapnia refers to an increased amount of carbon dioxide in the blood. 34
Four Stages of Hypoxia
Four stages of hypoxia need to be considered in examination of its effects on human pathophysiology. These four stages are divided by altitude. The first stage is the indifferent stage. The physiologic zone for this stage starts at sea level and extends to 10,000 ft. In this stage, the body reacts to the lessened availability of oxygen in the air with a slight increase in heart rate and ventilation. Night-vision deterioration occurs at 5000 ft. The second stage is the compensatory stage, which occurs from 10,000 to 15,000 ft. In this stage, the body attempts to protect itself against hypoxia. Increases in blood pressure, heart rate, and depth and rate of respiration occur. Efficiency and performance of tasks that require mental alertness become impaired in this stage. The third stage is the disturbance stage, which occurs between 15,000 and 20,000 ft. This stage is characterized by dizziness, sleepiness, tunnel vision, and cyanosis. Thinking becomes slowed, and muscle coordination decreases. The critical stage is the fourth stage of hypoxia. This stage occurs between 20,000 and 30,000 ft and features marked mental confusion and incapacitation followed by unconsciousness, usually within a few minutes. 20,21,23,26Table 5-1 contains a summary of the stages of hypoxia and its effects on humans.
Types of Hypoxia
On the basis of the physiologic effects elicited on the body, hypoxia can be divided into four different types: hypoxic hypoxia, hypemic hypoxia, stagnant hypoxia, and histotoxic hypoxia.
Hypoxic hypoxia is a deficiency in alveolar oxygen exchange. A reduction in PO 2 in inspired air or the effective gas exchange area of the lung may cause oxygen deficiency. The result is an inadequate oxygen supply to the arterial blood, which in turn decreases the amount of oxygen available to the tissues. 3,21,23 Decreased barometric pressure at high altitudes causes a reduction in the alveolar partial pressure of oxygen (PaO 2). The blood oxygen saturation, which is 98% at sea level, is reduced to 87% at 10,000 ft and 60% at 22,000 ft. This reduction in the amount of oxygen in the blood decreases the availability of the oxygen to the tissues and causes an impairment of body functions. 34 Hypoxic hypoxia is also referred to as altitude hypoxia because its primary cause is exposure to low barometric pressure. Hypoxic hypoxia interferes with gas exchange in two phases of respiration: ventilation and diffusion. During the ventilation phase, a reduction in PaO 2 may occur. Specific causes include breathing air at reduced barometric pressure, strangulation/respiratory arrest/laryngospasm, severe asthma, breath holding, hypoventilation, breathing gas mixtures with insufficient PO 2, and malfunctioning oxygen equipment at altitude. Causes of reduction in the gas exchange area include pneumonia, drowning, atelectasis, emphysema (chronic obstructive pulmonary disease), pneumothorax, pulmonary embolism, congenital heart defects, and physiologic shunting. Some causes of diffusion barriers are pneumonia and drowning. 3,21,23
Hypemic hypoxia is a reduction in the oxygen-carrying capacity of the blood. If the number of red blood cells per unit volume of blood is reduced, as from various types of anemia or from a loss of blood, the oxygen-carrying capacity and thus the oxygen content of the blood are reduced. 34 Even with normal ventilation and diffusion, cellular hypoxia can occur if the rate of delivery of oxygen does not satisfy metabolic requirements. 1,23,34 Hypemic hypoxia interferes with the transportation phase of respiration and causes a reduction in oxygen-carrying capacity. Specific causes of hypemic hypoxia include anemia, hemorrhage, hemoglobin abnormalities, use of drugs (e.g., sulfanilamides, nitrites), and intake of chemicals (e.g., cyanide, carbon monoxide). 23,31 Carbon monoxide is significant to air medical crews because it is present in the exhaust fumes of both conventional and jet-engine aircraft. It is also present in cigarette smoke and any fire or smoke situations. Carbon monoxide binds with hemoglobin 200 times more readily than does oxygen and displaces oxygen to form carboxyhemoglobin. 23,31
Stagnant hypoxia occurs when conditions result in reduced total cardiac output, pooling of the blood within certain regions of the body, a decreased blood flow to the tissues, or restriction of blood flow. 6,23 Stagnant hypoxia interferes with the transportation phase of respiration by reducing systemic blood flow. Specific causes include heart failure, shock, continuous positive-pressure breathing, acceleration (g forces), and pulmonary embolism. A reduction in regional or local blood flow may be caused by extremes of environmental temperatures, postural changes (prolonged sitting, bed rest, or weightlessness), tourniquets (restrictive clothing, straps), hyperventilation, embolism by clots or gas bubbles, and cerebral vascular accidents. 23,31
Histotoxic hypoxia (tissue poisoning) occurs when metabolic disorders or poisoning of the cytochrome oxidase enzyme system results in a cell’s inability to use molecular oxygen. 23,31 Histotoxic hypoxia interferes with the utilization phase of respiration because of metabolic poisoning or dysfunction. Specific causes include respiratory enzyme poisoning or degradation and the intake of carbon monoxide, cyanide, or alcohol. 23,31 Carbon monoxide can cause both hypemic and histotoxic hypoxia.
Effective Performance Time and Time of Useful Consciousness
These two terms are frequently used synonymously but are not interchangeable. Effective performance time (EPT) denotes the amount of time an individual is able to perform useful flying duties in an environment of inadequate oxygen. 20Time of useful consciousness (TUC) refers to the elapsed time from the point of exposure to an oxygen-deficient environment to the point at which deliberate function is lost. 6,29 EPT more accurately refers to critical (functional) performance than does TUC. With the loss of effective performance in flight, an individual is no longer capable of taking the proper corrective or protective action. 23 Thus, for air medical personnel the emphasis is on prevention.
In addition to altitude, factors that influence TUC are rate of ascent and an individual’s physical fitness, physical activity, temperature, individual tolerance, and self-imposed stresses, such as smoking, intake of alcohol and medication, and fatigue. 6 Another factor that dramatically reduces both EPT and TUC is rapid decompression, which occurs when a quick loss of cabin pressure occurs in a pressurized aircraft at high altitudes. On decompression at altitudes above 10,058 m (33,000 ft), an immediate reversal of oxygen flow in the alveoli takes place, caused by a higher P O2 within the pulmonary capillaries, which depletes the blood’s oxygen reserve and reduces the EPT at rest by up to 50%. Exercise also reduces the EPT considerably. 23,31,34Table 5-2 presents altitude and time of useful consciousness.
Altitude (in ft) | Time |
---|---|
18,000 and lower | 30 min |
25,000 | 3-5 min |
30,000 | 90 sec |
35,000 | 30-60 sec |
40,000 and higher | 15 sec or less |
Causes
Hypoxia has the three following causes: 1, high altitude; 2, hypoventilation; and 3, pathologic condition of the lung.
Characteristics
The onset of hypoxia may be gradual or insidious. Intellectual impairment occurs, demonstrated by slowed thinking, faulty memory of events, lessened immediate recall, delayed reaction time, and a tendency to fixate.
Early Signs and Symptoms
The individual symptoms of hypoxia can be identified in subjects under safe and controlled conditions in an altitude chamber. Once recognized, these symptoms do not vary dramatically in similar time exposures or among subjects. Hypoxia can be classified by objective signs (those perceived by an observer) or subjective symptoms (those perceived by the subject). 23 Signs and symptoms that appear on both lists in Box 5-1 may be seen by observers and recognized by the hypoxic subject when they occur. 3,11,18,21,35
BOX 5-1
Signs and Symptoms of Hypoxia
Objective Signs | Subjective Symptoms |
---|---|
Confusion | Confusion |
Tachycardia | Headache |
Tachypnea | Stupor |
Seizures | Insomnia |
Dyspnea | Change in judgment or personality |
Hypertension | |
Bradycardia | Dizziness |
Arrhythmias | Blurred vision |
Restlessness | Tunnel vision |
Slouching | Hot and cold flashes |
Unconsciousness | Tingling |
Hypotension (late) | Numbness |
Cyanosis (late) | Nausea |
Euphoria | Euphoria |
Belligerence | Anger |
Treatment
The treatment for hypoxia is administration of 100% oxygen. The type of hypoxia needs to be determined so that treatment can be administered accordingly. The following are required steps for transport team members:
1. Administer supplemental oxygen under pressure. Provision of adequate supplemental oxygen is the prime consideration in the treatment of hypoxia. Consideration must be given to the altitude and the cause of the oxygen deficiency. Equipment malfunction or altitude exposure above 12,192 m (40,000 ft) cannot be corrected without the addition of positive pressure. c The physiologic requirements for breathing are as follows:
Normal | Positive pressure |
Inspiration—active | Inspiration—passive |
Expiration—passive | Expiration—active |
The proper method of positive pressure breathing is as follows:

2. Monitor breathing. After a hypoxic episode, the resulting hyperventilation must be controlled to achieve complete recovery. A breathing rate of 12 to 16 breaths per minute or slightly lower aids recovery.
3. Monitor equipment. The most frequently reported causes of hypoxia are lack of oxygen discipline and equipment malfunction. A conscientious preflight check of equipment and frequent in-flight monitoring reduce this hazard. Inspection of oxygen equipment when hypoxia is suspected may detect its cause. Ground-transport team members must also conduct the same careful inspection of their equipment before and after transport to prevent any problems with their oxygen-delivery system during transport. Correction of a malfunction should bring immediate relief of the hypoxic condition. If treatment for hypoxia does not remedy the situation, oxygen contamination should be suspected. Use of an alternative oxygen source, such as the emergency oxygen cylinder or portable assembly, should be considered. Descent should be initiated as soon as possible, and the contents of the oxygen system should be analyzed.
The primary treatment of hypoxia for any patient being transported is prevention. The transport team must remember that the patient’s condition is already compromised and that stresses related to transport increase the risk of patient hypoxia unless the transport team continuously monitors the patient and accurately anticipates the oxygen needs of the patient during transport.
Hyperventilation
Hyperventilation at altitude is an important consideration for air medical personnel and also for the air medical patient. Hyperventilation is of concern because it produces changes in cellular respiration. Although the causes are unrelated, the symptoms of hyperventilation and hypoxia are similar and often result in confusion and inappropriate corrective procedures. Despite increased knowledge, training, and improved life-support equipment, both hypoxia and hyperventilation are hazards in flying and diving operations. 23Hyperventilation is an abnormal increase in the rate and depth of breathing that upsets the chemical balance of the blood31; it is commonly caused by psychologic stress (e.g., fear, anxiety, apprehensiveness, and anger) and environmental stress (e.g., hypoxia, pressure breathing, vibration, and heat). Certain drugs such as salicylates and female sex hormones also cause or enhance hyperventilation, and any condition that creates metabolic acidosis results in hyperventilation at high altitudes. 3,23,31
Treatment
At high altitudes, hyperventilation and hypoxia are treated in the same way because of similarities in the signs and symptoms. The following steps describe the treatment:
1. Administer 100% oxygen.
2. Begin positive-pressure breathing, which is the same as supplemental oxygen under pressure.
3. Regulate breathing and watch for hyperventilation.
4. Check equipment.
5. Descend.
The treatment for hyperventilation in the air medical patient is administration of oxygen. If treatment is successful, the amount of oxygen in the blood increases. Oxygen transfers from air to blood 20 times more slowly than carbon dioxide, and carbon dioxide transfers 20 times faster from blood to air than oxygen, which explains why the amount of carbon dioxide in the blood is directly associated with ventilation. When a patient is hyperventilating from anxiety, the act of putting a mask on the face to administer oxygen probably heightens the anxiety and increases tidal volume. Tidal volume must be reduced. 31 More favorable responses can be obtained by talking to patients to distract them, identifying causes of hyperventilation, and suggesting specific exercises to reduce respiratory rate. Several helpful exercises are:
1. The patient should count to 10 slowly while exhaling.
2. The patient should inhale and exhale only 10 times per minute.
3. Using a watch with a second hand, the patient should set a respiratory rate between 10 and 12 breaths per minute.
4. The air medical team member can provide counter pressure by suggesting isometric or active-passive exercises5 that cause the patient to hold the breath and reduce the respiratory rate.
Barometric Pressure
Boyle’s law states that at a constant temperature, the volume of a gas is inversely proportional to the pressure. On ascent, gases expand; on descent, gases contract. Therefore, trapped or partially trapped gases within certain body cavities (e.g., the gastrointestinal [GI] tract, lungs, skull, middle ear, sinuses, and teeth) expand in direct proportion to the decrease in pressure. 3,11,12,15,16,19,20,26,34
Middle Ear
The middle ear cavity is an air-filled space connected to the nasopharynx by the eustachian tube. The eustachian tube has a slit-like orifice at the throat end that allows air to vent outward more easily than inward. During ascent, air in the middle ear cavity expands but normally vents into the throat through the eustachian tube when a pressure differential of approximately 15 mm Hg has been reached. A mild fullness is usually detected but disappears as equalization occurs. This constitutes the passive process. 6 On descent, however, a different situation exists. The eustachian tube remains closed unless actively opened by muscle action or high positive pressure in the nasopharynx. If the eustachian tube opens, any existing pressure differential is immediately equalized. If the tube does not open regularly during descent, a pressure differential may develop. If this pressure differential reaches 80 to 90 mm Hg, the small muscles of the soft palate cannot overcome it, and either reascent or a maneuver that is not physiologic is necessary to open the tube. 10,22 On descent, equalization of pressure in the middle ear can be accomplished by performing the Valsalva’s maneuver, yawning, swallowing, moving the lower jaw, topical administration of vasoconstrictors, or use of a bag-valve mask. These procedures are examples of the active process.
Gum chewing is not recommended as a method of pressure equalization because it causes swallowing of air, thereby causing gastric distention and discomfort.
Barotitis Media
Barotitis media, frequently referred to as an ear block, results from failure of the middle ear space to ventilate when going from low to high atmospheric pressure (i.e., on descent). 11,22 Pressure in the middle ear becomes increasingly negative, and a partial vacuum is created. As the pressure differential increases, the tympanic membrane is depressed inward and becomes inflamed, and petechial hemorrhages develop. Blood and tissue fluids are drawn into the middle ear cavity, and if equalization with ambient pressure does not take place, perforation of the tympanic membrane occurs. Severe pain, tinnitus, and possibly vertigo and nausea can accompany acute barotitis. 22 Priority is placed on patient briefing before flight and adequate instructions for air medical crews. The ears should be cleared on descent with the methods previously described. Patients who are sleeping should be awakened before descent so they can clear their ears in the normal manner.
Patients with colds or upper respiratory tract infections must be closely monitored during both ascent and descent for swollen eustachian tubes, a condition that interferes with normal equalization procedures. 3,22 Air medical crew members with upper respiratory tract infections should not fly.
If an ear block occurs, mild vasoconstrictors should be administered early, and the plane should reascend to a higher altitude until symptoms lessen or the patient’s ear block clears. If patients have ear pain during ascent, which rarely occurs, air medical personnel should not have them execute a Valsalva’s maneuver because that would only aggravate the problem; instead, personnel should have them swallow or move their jaw muscles or administer to them a mild vasoconstrictor. 6 Either the Politzer’s bag or a source of compressed air may be used. A patient’s nose should be sprayed with a decongestant solution to attain maximal shrinkage of the mucosa. For the Politzer’s bag method, the olive tip is placed in one nostril, the nose is compressed between the air medical crew member’s fingers, and the patient is then instructed to say “kick, kick, kick” while the bag is squeezed, thereby increasing the pressure in the nasopharyngeal cavity to the point at which the eustachian tube is opened and the middle ear space ventilated. 11,22
In review, the treatment is as follows:
1. Patient performs Valsalva’s maneuver.
2. Crew member administers vasoconstrictor spray.
3. Crew member administers Politzer’s bag or bag-valve mask.
< div class='tao-gold-member'>
Only gold members can continue reading. Log In or Register a > to continue
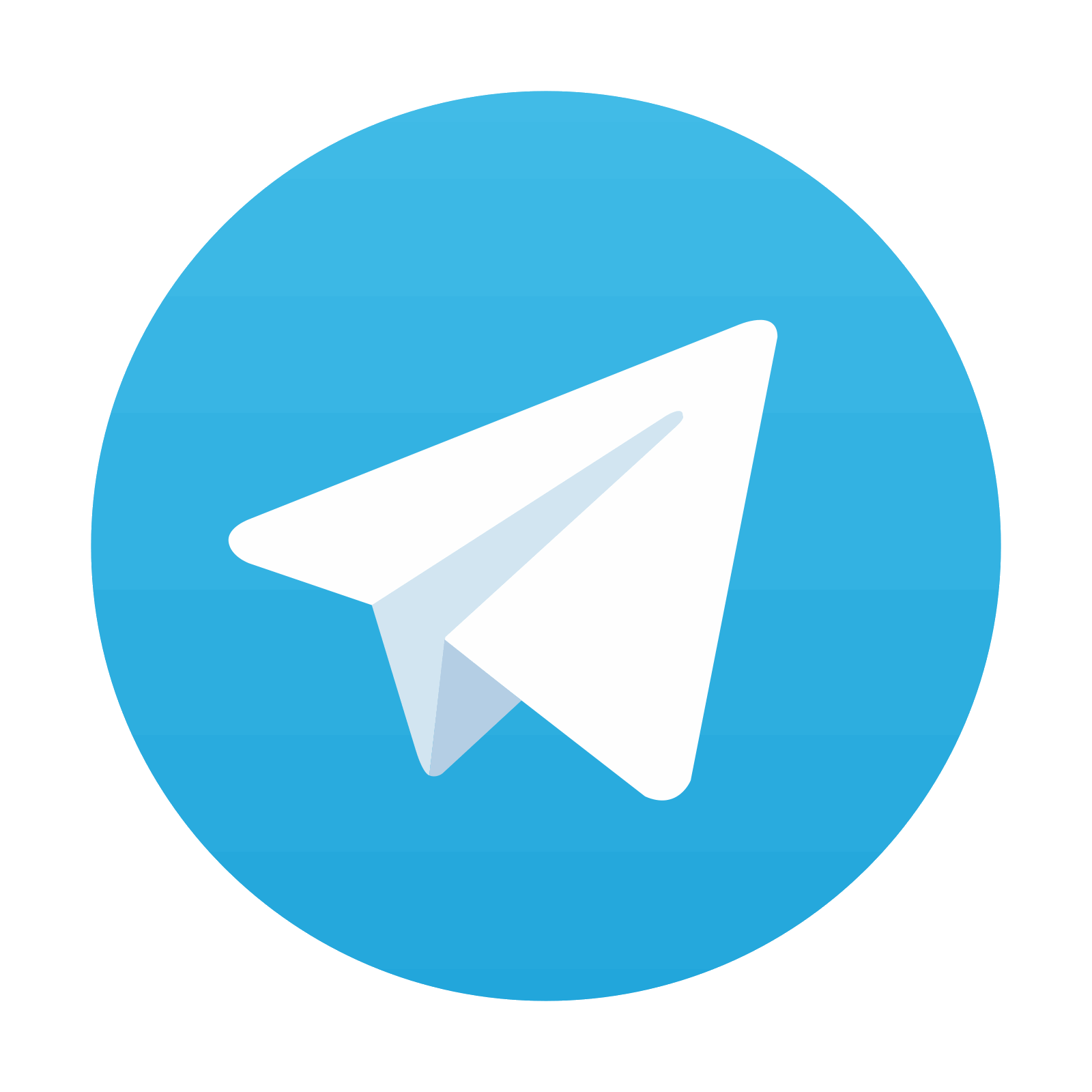
Stay updated, free articles. Join our Telegram channel
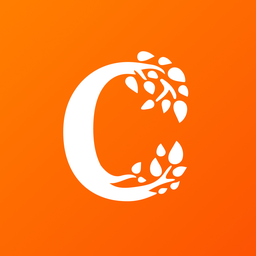
Full access? Get Clinical Tree
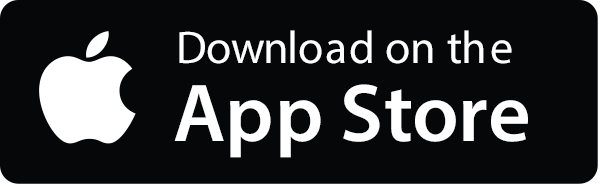
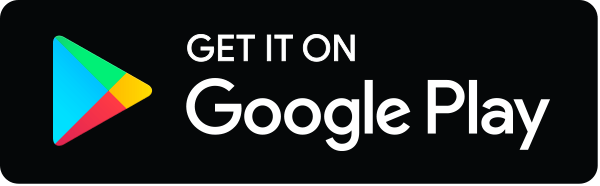
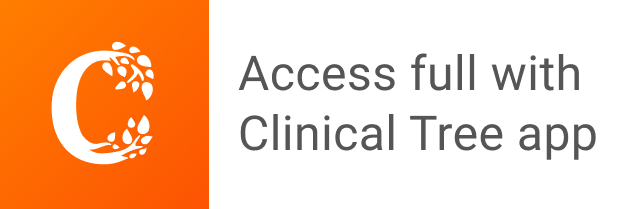