Renal filtration and reabsorption are susceptible to alterations by surgical illness and anesthesia. Autoregulation of renal blood flow (RBF) is effective over a wide range of mean arterial pressures (50 to 150 mm Hg). Autoregulation of urine flow does not occur, but a linear relationship between mean arterial pressure above 50 mm Hg and urine output is observed.
Renal medullary blood flow is low (2% of total RBF) but central to the kidneys’ ability to concentrate urine. During periods of reduced renal perfusion, the metabolically active medullary thick ascending limb may be especially vulnerable to ischemic injury.
The physiologic response to surgical stress invokes intrinsic mechanisms for sodium and water conservation. Renal cortical vasoconstriction causes a shift in perfusion toward juxtamedullary nephrons, a decrease in glomerular filtration rate, and retention of salt and water result.
The stress response may induce a decrease in RBF and glomerular filtration rate, causing afferent arteriolar vasoconstriction. If this situation is not reversed, ischemic damage to the kidney may result in acute renal failure (ARF).
Anesthetic-induced reductions in RBF have been described for many agents but are usually clinically insignificant and reversible. Likewise, anesthetic agents have not been shown to interfere with the renal response to physiologic stress.
Isolated ARF carries a mortality of up to 60% in surgical patients, with acute tubular necrosis being the cause of ARF in most of these patients.
Overall, there are no conclusive comparative studies demonstrating superior renal protection or improved renal outcome with general versus regional anesthesia.
Surgical patients with nondialysis-dependent chronic kidney disease are at higher risk of developing end-stage renal disease. The single most reliable predictor of new postoperative need for dialysis is preoperative renal insufficiency.
Maintaining adequate intravascular volume and hemodynamic stability with aggressive management of kidney hypoperfusion is a basic principle of anesthetic care to prevent acute kidney injury.
Urologic patients are often elderly, have numerous comorbidities, and require critical evaluation prior to any urologic procedure.
Combining epidural with general anesthetic techniques for some major surgeries may offer advantages for accelerated recovery, improved analgesia, and even better outcomes, but these techniques must be conducted with respect for other perioperative issues, including thromboprophylaxis for prevention of deep venous thrombosis.
Watchful waiting, minimally invasive principles, and technologic innovation (e.g., laparoscopy, robotics) have changed the favored approach to many kidney, bladder, and prostate disorders, in some cases reducing the number of high-risk surgeries, in others creating other safer less morbid alternate treatments.
Absorption of irrigating solution related most often to transurethral prostate or bladder tumor resections can cause “TUR syndrome,” a condition that, while becoming less common, has the potential to be serious and even life-threatening during several hours following surgery. Knowledge of specific concerns relevant to the different irrigating solutions, and vigilance of the anesthesiologist to factors that minimize absorption, recognition of signs and symptoms, and appropriate treatment are key to favorable outcomes with this condition.
Multimedia
Laparoscopic
Robotic Prostatectomy
INTRODUCTION AND CONTEXT
The kidney plays a central role in implementing and controlling a variety of homeostatic functions, including excreting metabolic waste products in the urine, while keeping extracellular fluid volume and composition constant. Acute kidney injury (AKI) can occur as a direct result of surgical or medical disease, prolonged reduction in renal oxygen delivery, nephrotoxin insult, or a combination of these three factors. The first part of this chapter reviews renal physiology and pathophysiologic states as they relate to anesthetic practice, and then addresses strategies for recognizing and managing patients at risk for AKI and renal failure. The second part describes current urologic procedures and their attendant anesthetic management issues.
RENAL ANATOMY AND PHYSIOLOGY
Gross Anatomy
The two normal kidneys are reddish-brown organs and are ovoid in outline, but the medial margin is deeply indented and concave at its middle (Fig. 49-1) where a wide, vertical cleft (the hilus) transmits structures entering and leaving the kidney. The hilus lies at approximately the level of the first lumbar vertebra. The kidneys lie in the paravertebral gutters, behind the peritoneum, with the right kidney lying slightly lower than the left one because of the presence of the liver. At its upper end, the ureter has dilated to give rise to the renal pelvis, which passes through the hilus into the kidney proper. There it is continuous with several short funnel-like tubes (calyces) that unite it with the renal parenchyma. The renal blood vessels lie anterior to the pelvis of the kidney, but some branches may pass posteriorly. Renal pain sensation is conveyed back to spinal cord segments T10 through L1 by sympathetic fibers. Sympathetic innervation is supplied by preganglionic fibers from T8 to L1. The vagus nerve provides parasympathetic innervation to the kidney, and the S2 to S4 spinal segments supply the ureters.
FIGURE 49-1. The gross anatomy (A) and internal structure of the genitourinary system and kidney. Internal organization of the kidney includes cortex and medulla regions and the vasculature (B). The nephron is the functional unit of the kidney (C). Plasma filtration occurs in the glomerulus (D); 20% of plasma that enters the glomerulus passes through the specialized capillary wall into the Bowman capsule and enters the tubule to be processed and generate urine. PCT, proximal convoluted tubule, DCT, distal convoluted tubule. (From: http://www.incontinenceaid.com/files/images/Urinary_system_components.jpg [A]; B; http://www.pathology.vcu.edu/education/PathLab/pages/renalpath/ rpsrhome.htm from Lecture 1 (modified with permission) [C].)
The kidneys are enclosed by a thick, fibrous capsule, itself surrounded by a fatty capsule that fills the space inside the loosely applied renal (Gerota’s) fascia. The developing kidney is first formed in the pelvis and then ascends to its final position on the posterior abdominal wall. During its ascent, the kidney receives blood supply from several successive sources, such that an accessory renal artery from the aorta may be found entering the lower pole of the kidney. When first formed, the rudimentary kidneys are close together and may fuse to give rise to a horseshoe kidney. This organ is unable to ascend, “held in place” by the inferior mesenteric artery, and thus when present it remains forever a pelvic organ.
The bladder is located in the retropubic space and receives its innervation from sympathetic nerves originating from T11 to L2, which conduct pain, touch, and temperature sensations, whereas bladder stretch sensation is transmitted via parasympathetic fibers from segments S2 to S4. Parasympathetics also provide the bladder with most of its motor innervation.
The prostate, penile urethra, and penis also receive sympathetic and parasympathetic fibers from the T11 to L2 and S2 to S4 segments, respectively. The pudendal nerve provides pain sensation to the penis via the dorsal nerve of the penis. Sensory innervation of the scrotum is via cutaneous nerves, which project to lumbosacral segments, whereas testicular sensation is conducted to lower thoracic and upper lumbar segments.
Ultrastructure
Inspection of the cut surface of the kidney reveals the paler cortex, adjacent to the capsule, and the darker, conical pyramids of the renal medulla (Fig. 49-1). The pyramids are radially striated and are covered with cortex, extending into the kidney as the renal columns. Collecting tubules from each lobe of the kidney (pyramid and its covering of cortex) discharge urine into the calyceal system via renal papillae at the entrance of each pyramid into the calyx proper. These collecting tubules originate deep within the radial striations (medullary rays) of the kidney and convey urine formed in the structural units of the kidneys, the nephrons. The parenchyma of each kidney contains approximately 1 × 106 tightly packed nephrons, each one consisting of a tuft of capillaries (the glomerulus) invaginated into the blind, expanded end (glomerular corpuscle) of a long tubule that leaves the renal corpuscle to form the proximal convoluted tubule in the cortex. This leads into the straight tubule, which loops down into the medullary pyramid (loop of Henle) and hence back to the cortex to become continuous with the distal convoluted tubule. This then opens into a collecting duct that is common to a number of nephrons and passes through the pyramid to enter the lesser calyx at the papilla. It is in these parts of the nephron (proximal tubule, loop of Henle, distal tubule, and collecting duct) that urine is formed, concentrated, and conveyed to the ureters. The distal convoluted tubule comes into very close contact with the afferent glomerular arteriole, and the cells of each are there modified to form the juxtaglomerular apparatus, a complex physiologic feedback control mechanism contributing in part to the precise control of intra- and extrarenal hemodynamics that is a hallmark feature of the normally functioning kidney.
As is the case for the renal tubules, the vasculature of the kidney is highly organized. The renal artery enters the kidney at the hilum and then divides many times before producing the arcuate arteries that run along the boundary between cortex and outer medulla. Interlobular arteries branch from arcuate arteries toward the outer kidney surface, giving rise as they pass through the cortex to numerous afferent arterioles, each leading to a single glomerular capillary tuft. The barrier where filtration from the vascular to tubular space within the glomerulus occurs is highly specialized and includes fenestrated negatively charged capillary endothelial cells and tubular epithelial cells (podocytes) separated by a basement membrane. Normally, selective permeability permits approximately 25% of the plasma elements to pass into the Bowman capsule; only cells and proteins >60 to 70 kDa cannot cross. However, abnormalities of this barrier can occur with diseases that may permit filtration of much larger proteins and even red blood cells; these changes manifest as the nephrotic syndrome (proteinuria >3.5 g/24 hr) or glomerulonephritis (hematuria and proteinuria). The glomerular capillaries exit the Bowman capsule and merge to form the efferent arteriole and peritubular capillaries that nourish the tubules. The renal vasculature is unusual in having this arrangement of two capillary beds joined in series by arterioles. Blood supply to the entire tubular system comes from the glomerular efferent arteriole, which branches into an extensive capillary network. Some of these peritubular capillaries, the vasa recta, descend deep into the medulla to parallel the loops of Henle. The vasa recta then return in a cortical direction with the loops, join other peritubular capillaries, and empty into the cortical veins.
Correlation of Structure and Function
Because renal tissue makes up only 0.4% of body weight but receives 25% of cardiac output, the kidneys are by far the most highly perfused major organs in the body, and this facilitates plasma filtration at rates as high as 125 to 140 mL/min in adults. The functions of the kidney are many and varied, including waste filtration, endocrine and exocrine activities, immune and metabolic functions, and maintenance of physiologic homeostasis. As well as tight regulation of extracellular solutes such as sodium, potassium, hydrogen, bicarbonate, and glucose, the kidney also generates ammonia and glucose and eliminates nitrogenous and other metabolic wastes including urea, creatinine, and bilirubin. Finally, circulating hormones secreted by the kidney influences red blood cell generation, calcium homeostasis, and systemic blood pressure.
The kidney fulfills its dual roles of waste excretion and body fluid management by filtering large amounts of fluid and solutes from the blood and secreting waste products into the tubular fluid. Filtration and reabsorption are affected by comorbid disease, surgery, and anesthesia and are the focus of the next section.
Glomerular Filtration
Production of urine begins with water and solute filtration from plasma flowing into the glomerulus via the afferent arteriole. The glomerular filtration rate (GFR) is a measure of glomerular function expressed as milliliters of plasma filtered per minute. The ultrafiltration constant (Kf ) is directly related to glomerular capillary permeability and glomerular surface area. The two major determinants of filtration pressure are glomerular capillary pressure (PGC) and glomerular oncotic pressure (pGC). PGC is directly related to renal artery pressure and is heavily influenced by arteriolar tone at points upstream (afferent) and downstream (efferent) from the glomerulus. An increase in afferent arteriolar tone, as occurs with intense sympathetic or angiotensin II stimulation, causes filtration pressure and GFR to fall. Milder degrees of sympathetic or angiotensin activity cause a selective increase in efferent arteriolar tone, which tends to increase filtration pressure and GFR. The pGC is directly dependent on plasma oncotic pressure. Afferent arteriolar dilatation enhances GFR by increasing glomerular flow, which in turn elevates glomerular capillary pressure.
Autoregulation of Renal Blood Flow and Glomerular Filtration Rate
Renal blood flow (RBF) autoregulation maintains relatively constant rates of RBF and glomerular filtration over a wide range of arterial blood pressure. Renal autoregulation of blood flow and filtration is accomplished primarily by local feedback signals that modulate glomerular arteriolar tone to protect the glomeruli from excessive perfusion pressure (Fig. 49-2).
FIGURE 49-2. Renal blood flow (RBF) autoregulation maintains RBF and glomerular filtration rate (GFR) relatively constant with changes in systolic blood pressure from about 80 to 200 mm Hg. (From: http://www2.kumc.edu/ki/physiology/course/two/2_8.htm, with permission.)
In health, autoregulation of RBF is effective over a wide range of systemic arterial pressures. Several mechanisms for regulating blood flow to the glomerulus have been described, and all involve modulation of afferent glomerular arteriolar tone. The myogenic reflex theory holds that an increase in arterial pressure causes the afferent arteriolar wall to stretch and then constrict (by reflex); likewise, a decrease in arterial pressure causes reflex afferent arteriolar dilatation. The other proposed mechanism of RBF autoregulation is a phenomenon called tubuloglomerular feedback, which is also responsible for autoregulation of GFR.
Tubuloglomerular feedback allows the composition of distal tubular fluid to influence glomerular function through actions involving the juxtaglomerular apparatus. When RBF falls, the concomitant decrease in GFR results in less chloride delivery to the juxtaglomerular apparatus, which causes the afferent arteriole to dilate. Glomerular flow and pressure then increase, and GFR returns to previous levels. Chloride also acts as the feedback signal for control of efferent arteriolar tone. When GFR falls, declining chloride delivery to the juxtaglomerular apparatus triggers release of renin, which ultimately causes the formation of angiotensin II. In response to angiotensin, efferent arteriolar constriction increases glomerular pressure, which increases glomerular filtration. It is important to realize that autoregulation of urine flow does not occur, and that above a mean arterial pressure of 50 mm Hg there is a linear relationship between mean arterial pressure and urine output.
Tubular Reabsorption of Sodium and Water
Active, energy-dependent reabsorption of sodium begins almost immediately as the glomerular filtrate enters the proximal tubule. Here, an adenosine triphosphatase pump drives the sodium into tubular cells while chloride ions passively follow. Glucose, amino acid, and other organic compound reabsorption are strongly coupled to sodium in the proximal tubule. Normally, the proximal tubule reabsorbs two-thirds of the filtered sodium, but no active sodium transport occurs in the loop of Henle until the medullary thick ascending limb is reached. Cells of the medullary thick ascending limb are metabolically active in their role of reabsorbing sodium and chloride and have a high oxygen consumption compared with the thin portions of the descending and ascending limbs.
Reabsorption of water is a passive, osmotically driven process tied to the reabsorption of sodium and other solutes. Water reabsorption also depends on peritubular capillary pressure; high capillary pressure opposes water reabsorption and tends to increase urine output. The proximal tubule reabsorbs approximately 65% of filtered water in an isosmotic fashion with sodium and chloride. The descending limb of the loop of Henle allows water to follow osmotic gradients into the renal interstitium. However, the thin ascending limb and medullary thick ascending limb are relatively impermeable to water and play a key role in the production of concentrated urine. Only 15% of filtered water is reabsorbed by the loop of Henle; the remaining filtrate volume flows into the distal tubule. There, and in the collecting duct, water reabsorption is controlled entirely by antidiuretic hormone (ADH) secreted by the pituitary gland. Conservation of water and excretion of excess solute by the kidneys would be impossible without the ability to produce concentrated urine. This is accomplished by establishing a hyperosmotic medullary interstitium and regulation of water permeability of the distal tubule and collecting duct via the action of ADH.
ADH increases the water permeability of the collecting ducts and allows for passive diffusion of water (under considerable osmotic pressure) back into the circulation. The posterior pituitary gland releases ADH in response to an increase in either extracellular sodium concentration or extracellular osmolality. In addition, ADH release can be triggered by an absolute or relative reduction in intravascular fluid volume. The arterial baroreceptors are activated when hypovolemia leads to a decrease in blood pressure, whereas atrial receptors are stimulated by a decline in atrial filling pressure. Both of these circulatory reflex systems stimulate release of ADH from the pituitary and cause retention of water by the kidney in an effort to return the intravascular volume toward normal. ADH also causes renal cortical vasoconstriction when it is released in large amounts, such as during the physiologic stress response to trauma, surgery, or other critical illness. This induces a shift of RBF to the hypoxia-prone renal medulla.
The Renin–Angiotensin–Aldosterone System
Renin release by the afferent arteriole may be triggered by hypotension, increased tubular chloride concentration, or by sympathetic stimulation. Renin enhances angiotensin II production, which in turn induces renal efferent arteriolar vasoconstriction. Angiotensin II also promotes ADH release from the posterior pituitary, sodium reabsorption by the proximal tubule, and aldosterone release by the adrenal medulla. Aldosterone stimulates the distal tubule and collecting duct to reabsorb sodium (and water), resulting in intravascular volume expansion. Sympathetic nervous system stimulation may also directly cause release of aldosterone. This leads to renal cortical vasoconstriction, a decrease in GFR, and salt and water retention.
Renal Vasodilator Mechanisms
Opposing the saline retention and vasoconstriction observed in stress states are the actions of atrial natriuretic peptide (ANP), nitric oxide, and the renal prostaglandin system. ANP is released by the cardiac atria in response to increased stretch under conditions of volume expansion. Both natriuresis and aquaresis increase as ANP blocks reabsorption of sodium in the distal tubule and collecting duct. ANP also increases GFR, causes systemic vasodilatation, inhibits the release of renin, opposes production and action of angiotensin II, and decreases aldosterone secretion.1,2 Likewise, nitric oxide produced in the kidney opposes the renal vasoconstrictor effects of angiotensin II and the adrenergic nervous system, promotes sodium and water excretion, and participates in tubuloglomerular feedback.3
Prostaglandins are produced by the kidney as part of a complex system that modulates RBF and opposes the actions of ADH and the renin–angiotensin–aldosterone system.4 Stress states, renal ischemia, and hypotension stimulate the production of renal prostaglandins through the enzymes phospholipase A2 and cyclooxygenase. Prostaglandins produced by cyclooxygenase activity cause dilatation of renal arterioles (antiangiotensin II), whereas their distal tubular effects result in an increase in sodium and water excretion (anti-ADH and aldosterone). The renal prostaglandin system is important in maintaining RBF and sodium and water excretion during times of high physiologic stress and poor renal perfusion.4
CLINICAL ASSESSMENT OF THE KIDNEY
Most agree that measures such as urine output correlate poorly with perioperative renal function5; however, much about the kidneys can be learned from knowing how effectively they clear circulating substances and inspection of the urine (i.e., urinalysis).
Renal Function Tests
Filtration is a useful method to clinically assess kidney function. As a key indicator of disease, knowledge of limited filtration capacity is important to guide drug dosing for agents cleared by the kidneys and helps with preoperative risk stratification. Also, acute declines in filtration capacity indicate kidney injury and predict a more complicated clinical course.2 GFR, as previously mentioned, refers to the plasma volume filtered per unit time by the kidneys, and normal values range from 90 to 140 mL/min. Normal GFRs relate to the patient age, size, and gender. In general, GFR declines 10% per decade after age 30 and is approximately 10 mL/min higher in men than women. A GFR below 60 mL/min meets criteria for chronic kidney disease (CKD) and is considered impaired, while values lower than 15 mL/min are often associated with uremic symptoms and may require dialysis.
An “ideal” substance to assess GFR through its clearance from the circulation must have specific properties, including a steady supply, free filtration, and no tubular reabsorption or excretion; ideally, it is also cheap and easy to measure. Unfortunately, the perfect ideal substance is yet to be identified. The “gold standard” GFR tools involve expensive and cumbersome measurements (e.g., inulin, 51Cr-EDTA or 99Tc-DTPA clearance), while the most practical and inexpensive test involves an imperfect “ideal” substance, creatinine. However, despite creatinine’s limitations, its relatively steady supply from muscle metabolism, modest tubular secretion, and proven usefulness in numerous clinical settings make it the most used renal filtration marker currently available. Although more ideal substances and other “early biomarkers” of AKI are being evaluated as clinical tools, current candidates (e.g., cystatin C) have yet to replace creatinine.
Estimates of GFR (eGFR) can be made by determining creatinine clearance (CrCl) from urine and blood creatinine tests. In stable, critically ill patients, 2-hour urine collections are sufficient to calculate CrCl,6 using the following formula:
where UCr = urine creatinine, V = total volume of urine collected, PCr = plasma creatinine, time = collection time.
However, if patient characteristics are known, GFR can also be estimated from a single steady-state serum creatinine value. Notably, predictive formulas are developed using data from stable (nonsurgical) populations, and factors such as fluid shifts, hemodilution, and hemorrhage may add an “unsteadiness” to perioperative eGFR using serum creatinine.
Nonetheless, serum creatinine remains, so far, an unsurpassed perioperative tool, particularly to reflect trends of change in renal filtration and to predict outcome, even during the perioperative period.7–9 Of the predictive formulas, the Cockroft–Gault equation is one of the oldest and most durable.10 The Cockroft–Gault equation uses patient gender, age (years), weight (kg), and serum creatinine (mg/dL):
More recently, a method developed from the Modification of Diet in Renal Disease (MDRD) study that adds other factors including ethnicity (black vs. nonblack) to Cockroft–Gault equation has gained popularity.11
An abbreviated MDRD formula is available that can estimate GFR measured in milliliters per minute per 1.73 m2:
However, even a detailed MDRD eGFR under ideal conditions sometimes correlates poorly with a gold standard-determined GFR, with more than a 30% error in 10% of patients, and 2% deviating more than 50%.11
Some consensus definitions for significant perioperative renal dysfunction exist. For example, the Society of Thoracic Surgeons defines postoperative AKI as either a new requirement for dialysis or a rise in serum creatinine to >2 mg/dL involving at least a 50% increase in serum creatinine above baseline.12 Another definition requires a creatinine rise of >25% or 0.5 mg/dL (44 mmol/L) within 48 hours.13 The Acute Dialysis Quality Initiative (ADQI) Group definition for critically ill patients grades AKI by an acute creatinine rise of 50% as risk, 100% as injury, or 200% as failure (the RIFLE criteria).14 The Acute Kidney Injury Network (AKIN) definition, a 1.5-fold or 0.3 mg/dL (≥26.4 mmol/L) creatinine rise within a 48-hour period or more than 6 hours of oliguria (>0.5 mL/ kg/hr), is a modification of its RIFLE predecessor.14,15 Notably, serum creatinine does not usually rise significantly until GFR rates fall below 50 mL/min, so preoperative serum creatinine may be normal in patients even with some degree of renal dysfunction (Fig. 49-3).
FIGURE 49-3. The nonlinear relationship between changes in renal filtration and serum creatinine level means that a large reduction (e.g., 75%, 120 to 30 mL/min) in glomerular filtration rate (GFR) may be associated with a modest rise in serum creatinine. Proportional reductions in GFR and (approximate) nephron loss (x axis) have an inverse logarithmic relationship with serum creatinine concentration (y axis). (Modified from: Faber MD, Kupin WL, Krishna G, et al. In: Lazarus JM, Brenner BM, eds. Acute Renal Failure: The differential diagnosis of ARF. 3rd ed. New York, NY: Churchill Livingstone, 1993, 133, with permission.)
Blood urea nitrogen (BUN) is sometimes used to assess renal function but possesses few of the characteristics of an ideal substance. Tubular urea transport changes with some conditions (e.g., dehydration), and urea generation can be highly variable, particularly during the postoperative period (i.e., catabolic state). In addition, hemodilution (e.g., cardiopulmonary bypass [CPB]) may affect circulating BUN levels.
Urinalysis and Urine Characteristics
Urine inspection can reveal abnormal cloudiness, color, and unexpected odors. Detailed descriptions of urine examination are available16; therefore, only a summary is provided here. Cloudy urine is due to suspended elements such as white or red blood cells and/or crystals. Lightly centrifuged urine sediment will normally contain 80 ± 20 mg of protein per day and up to two red blood cells per high-power field (400×); higher levels of red blood cells or protein reflect abnormal kidney function. Urine protein electrophoresis can differentiate proteinuria from a glomerular (filtering), tubular (reuptake), overflow (supply that saturates the reuptake system), or tissue (e.g., kidney inflammation) abnormality.17 In contrast, color changes reflect dissolved substances; this occurs most commonly with dehydration, but other causes include food colorings, drugs, and liver disease (e.g., bilirubin). Unusual odors are less common but can also be diagnostic (e.g., maple syrup urine disease). Chromogenic “dipstick” chemical tests can determine urine pH and provide a semiquantitative analysis of protein, blood, nitrites, leukocyte esterase, glucose, ketones, urobilinogen, and bilirubin. In addition, microscopy can identify crystals, cells, tubular casts, and bacteria.
Urine specific gravity (the weight of urine relative to distilled water) normally ranges between 1.001 and 1.035, and can be used as a surrogate for osmolarity (normal 50 to 1,000 mOsm/kg), with 1.010 reflecting a specific gravity similar to that of plasma. High specific gravity (>1.018) implies preserved renal concentrating ability, unless high levels of glucose, protein, or contrast dye injection have raised specific gravity without significantly changing osmolarity.
Although poor urine output (e.g., <400 mL urine/24 hr) may reflect hypovolemia or impending prerenal renal failure, a majority of perioperative AKI episodes develop in the absence of oliguria.1 The normal response to hypovolemia is renal solute retention; fluid and electrolyte retention produces a concentrated urine with a low sodium content (<20 mEq/L). In contrast, impaired concentrating ability due to AKI causes urine to approach plasma osmolarity (isosthenuria) with a higher sodium content (>40 mEq/L). The kidneys’ ability to retain electrolytes is also reflected in the fractional excretion of sodium (FENa), a test that uses a spot sample of urine and blood to compare sodium and creatinine excretion; this test can be useful to distinguish hypovolemia and renal injury:
where UNa = urine sodium, PNa = plasma sodium, UCr = urine creatinine, and PCr = plasma creatinine.
FENa <1% implies that sodium is being normally conserved while values above 1% are consistent with acute tubular necrosis (ATN).
PERIOPERATIVE NEPHROLOGY
Pathophysiology
Altered renal function can be thought of as a clinical continuum ranging from the normal compensatory changes seen during stress to frank renal failure. Clinically, there is considerable overlap between compensated and decompensated renal dysfunctional states. The kidney under stress reacts in a predictable manner to help restore intravascular volume and maintain blood pressure. The sympathetic nervous system reacts to trauma, shock, or pain by releasing norepinephrine, which acts much like angiotensin II on the renal arterioles. Norepinephrine also activates the renin–angiotensin–aldosterone system and causes ADH release. The net result of modest activity of the stress response system is a shift of blood flow from the renal cortex to the medulla, avid sodium and water reabsorption, and decreased urine output. A more intense stress response may induce a decrease in RBF and GFR by causing afferent arteriolar constriction. If this extreme situation is not reversed, ischemic damage to the kidney may result, and AKI may become clinically manifest.
Electrolyte Disorders
Disorders of Sodium Balance
Hyponatremia is the most commonly occurring electrolyte disorder18,19 (see also Chapter 14). Symptoms rarely occur unless sodium values are <125 mmol/L, and these include a spectrum ranging from anorexia, nausea, and lethargy to convulsions, dysrhythmias, coma, and even death due to osmotic brain swelling.20–22 Hyponatremia may occur in the setting of an expanded (e.g., transurethral resection [TUR] syndrome), normal, or contracted extracellular fluid volume, and volemic status and urinary sodium concentration are key markers in differentiating the large number of potential causes of hyponatremia. If water excess is a reason for hyponatremia, dilute urine (sodium >20 mmol/L) is expected. Conversely, avid renal sodium retention (urine sodium <20 mmol/L) suggests sodium loss as a cause. If hyponatremia is acute, the risk of neurologic complications is higher, and cautious treatment is indicated to prevent cerebral edema and seizures. This should be accomplished with intravenous hypertonic saline and furosemide to enhance water excretion and prevent sodium overload (see transurethral resection syndrome section).
Hypernatremia (serum sodium >145 mmol/L) is generally the result of sodium gain or water loss, most commonly the latter. Dehydration of brain tissue can cause symptoms ranging from confusion to convulsions and coma. In cases of hypernatremia, laboratory studies often show evidence of hemoconcentration (increased hematocrit and serum protein concentrations). In addition, urine output is usually low (<500 mL/day) and hyperosmolar (>1,000 mOsm), with very low urinary sodium concentration and evidence of prerenal failure (elevations of BUN and serum creatinine). Occasionally, the urine is not maximally concentrated, suggesting an osmotic diuresis or an intrinsic renal disorder such as diabetes insipidus. The primary goal of treatment is restoration of serum tonicity, which can be achieved with isotonic or hypotonic parenteral fluids and/or diuretics unless irreversible renal injury is present, in which situation dialysis may be necessary.
Disorders of Potassium Balance
Even minor variations in serum potassium concentration can lead to symptoms such as skeletal muscle weakness, gastrointestinal ileus, myocardial depression, malignant ventricular dysrhythmias, and asystole. Nearly 98% of total body potassium is intracellular. Circulating potassium levels are tightly controlled via renal and gastrointestinal excretion and reabsorption, but potassium also moves between the intra- and extracellular compartments under the influence of insulin and b2-adrenoceptors. In the kidney, 70% of potassium reabsorption occurs in the proximal tubule and another 15% to 20% in the loop of Henle. The collecting duct is responsible for potassium excretion under the influence of aldosterone.
Hypokalemia may be due to a net potassium deficiency or transfer of extracellular potassium to the intracellular space. Notably, total body depletion may exist even with normal extracellular potassium levels (e.g., diabetic ketoacidosis). Causes of hypokalemia include extrarenal loss (e.g., vomiting, diarrhea), renal loss (impaired processing due to drugs, hormones, or inherited renal abnormalities), potassium shifts between the extra- and intracellular spaces (e.g., insulin therapy), and, occasionally, inadequate intake. Clinical manifestations of hypokalemia include ECG changes (flattened T waves—“no pot, no T”, U waves, prodysrhythmic state) and skeletal muscle weakness. Hypokalemia treatment involves supplementation with intravenous or oral potassium; however, overly rapid potassium intravenous administration should be avoided because it can cause hyperkalemic cardiac arrest.
If a patient has hyperkalemia (elevated serum potassium level >5.5 mEq/L), it is important to consider the duration of the condition as chronic hyperkalemia is far better tolerated than an acute rise. Other than laboratory artifacts (e.g., hemolyzed sample), causes of hyperkalemia include abnormal kidney excretion, abnormal cellular potassium release, or abnormal distribution between the intracellular and the extracellular space.
Disorders of Calcium, Magnesium, and Phosphorus
Most of a grown adult’s 1 to 2 kg of calcium is in bone (98%), with the remaining 2% in one of the three forms: Ionized, chelated, or protein-bound. Normal serum calcium values range between 8.5 and 10.2 mg/dL, but only the ionized fraction (50%) is biologically active and precisely regulated. Ionized extracellular calcium concentration (iCa++) is controlled by the combined actions of parathyroid hormone (PTH), calcitonin, and vitamin D and further modulated by dietary and environmental factors. Hypocalcemia due to reduced serum protein levels is physiologically unimportant. Extracellular magnesium represents only 0.3% of total (mainly intracellular) stores, making normal serum levels (1.6 to 2.2 mg/dL) a poor reflection of total body magnesium. Phosphorus is a major intracellular anion that plays a role in regulation of glycolysis, ammoniagenesis, and calcium homeostasis and is an essential component of adenosine triphosphate and red blood cell 2,3-diphosphoglyceric acid synthesis.
The clinical manifestations of hypocalcemia include cramping, digital numbness, laryngospasm, carpopedal spasm, bronchospasm, seizures, and respiratory arrest. A positive Chvostek sign (facial muscle twitching in response to tapping the facial nerve) or Trousseau sign (carpal spasm induced by brachial artery occlusion) are the classic hallmarks of hypocalcemia but in practice are often absent. Mental status changes, including irritability, depression, and impaired cognition, may also occur. Cardiac manifestations include QT interval shortening and dysrhythmias.
Hypocalcemia may be due to several mechanisms, including a decrease in PTH secretion or action, reduced vitamin D synthesis or action, resistance of bone to PTH or vitamin D effects, or calcium sequestration. Citrate used for regional anticoagulation with dialysis can also cause hypocalcemia and may also lead to hypomagnesemia from decreased PTH secretion. Parathyroidectomy during neck surgery reduces PTH levels and is a common cause of acquired hypoparathyroidism.
Clinical symptoms of hypercalcemia correlate with its acuity and include constipation, nausea and vomiting, drowsiness, lethargy, weakness, stupor, and coma. Cardiovascular manifestations may include hypertension, shortened QT interval, heart block, and other dysrhythmias. The most frequent causes of hypercalcemia are primary hyperparathyroidism and malignancy. Other causes include thiazide (increased renal calcium reabsorption) or lithium (inhibits PTH release) therapy and rarer medical conditions including granulomatous disease, thyrotoxicosis, and multiple endocrine neoplasia (MEN) types I and II.
Hypomagnesemia (<1.6 mg/dL) may sometimes be asymptomatic, but clinically important problems can and do manifest, including neuromuscular, cardiac, neurologic, and related electrolytic (hypokalemia and hypocalcemia) abnormalities. Causes of hypomagnesemia can be divided in four broad categories: Decreased intake, gastrointestinal loss, renal loss, and redistribution. Nutritional hypomagnesemia can result from malabsorption syndromes in patients receiving parenteral nutrition, and it is also present in 25% of alcoholics. Redistribution occurs with acute pancreatitis, administration of catecholamines, and in “hungry bone syndrome” after parathyroidectomy.10 Magnesium can be supplemented orally or via the parenteral route.
Clinical manifestations of hypermagnesemia (>4 to 6 mg/dL) are serious and potentially fatal. Minor symptoms include hypotension, nausea, vomiting, facial flushing, urinary retention, and ileus. In more extreme cases, flaccid skeletal muscular paralysis, hyporeflexia, bradycardia, bradydysrhythmias, respiratory depression, coma, and cardiac arrest may occur. Hypermagnesemia generally occurs in two clinical settings: Compromised renal function (GFR <20 mL/min) and excessive magnesium intake (e.g., excessive intravenous therapy in preeclampsia). Although mild hypermagnesemia in the setting of normal renal function can be treated with supportive care and withdrawal of the cause, in some cases dialysis is necessary.
Hypophosphatemia is clinically more important than hyperphosphatemia and can result in symptoms including muscle weakness, respiratory failure, and difficulty in weaning critically ill patients from mechanical ventilation when serum levels are <0.32 mmol/L. In addition, low phosphate levels may diminish oxygen delivery to tissues and rarely cause hemolysis. Hypophosphatemia can result from intracellular redistribution (from catecholamine therapy), from inadequate intake or absorption secondary to alcoholism or malnutrition, or from increased renal or gastrointestinal losses.12 Intravenous and oral supplementation can be used to treat hypophosphatemia.
Hyperphosphatemia (>5 mg/dL) is generally related to accompanying hypocalcemia although increased phosphate levels may also lead to calcium precipitation and decreased intestinal calcium absorption.13,14 Significantly elevated serum phosphate levels are most commonly due to reduced excretion from renal insufficiency but can also result from excess intake or redistribution of intracellular phosphorus. Treatment of chronic hyperphosphatemia includes dietary phosphate restriction and oral phosphate binders.
Acid–Base Disorders
The primary determinant of serum pH is the balance between plasma bicarbonate (HCO3−) concentration and the PCO2 in the extracellular space. Acid–base homeostasis involves tight regulation of HCO3− and PaCO2. Primary extracellular pH derangements due to abnormal bicarbonate reabsorption and proton (H+) elimination by the kidney lead to metabolic acidosis or alkalosis, while factors that abnormally affect respiratory drive influence PaCO2, leading to respiratory acidosis or alkalosis. Because combined problems are often seen in perioperative critically ill patients, an approach to both “pure” and “mixed” acid–base disorders is presented here.
Metabolic Acidosis
The anion gap (AG) represents the total serum concentration of unmeasured anions and can be calculated as AG = (Na+ + K+) − (HCO3− + Cl−). It allows differentiation of the causes of metabolic acidosis into normal AG (8 ± 4) and increased AG (>16 mmol/L) varieties. Conditions that cause an increase in negatively charged ions other than bicarbonate and chloride (e.g., lactate, salicylate) increase the AG. In contrast, non-AG metabolic acidosis results from renal or gastrointestinal HCO3− loss and is associated with high chloride levels (hyperchloremic metabolic acidosis). The usual compensatory response to all types of metabolic acidoses is hyperventilation, which leads to a partial pH correction toward normal. Winter’s formula predicts expected PaCO2 for a metabolic acidosis as follows: PaCO2 = (1.5 × HCO3−) + 8.
Metabolic Alkalosis
Metabolic alkalosis is a common primary acid–base disturbance associated with increased plasma HCO3−. Increased extracellular HCO3− is due to a net loss of H+ and/or addition of HCO3−. The most common cause of metabolic alkalosis is gastrointestinal acid loss due to vomiting or nasogastric suctioning; the resulting hypovolemia leads to secretion of renin and aldosterone and enhanced absorption of HCO3−. Thiazides and loop diuretics both induce a net loss of chloride and free water and can cause a volume “contraction” alkalosis.
Respiratory Acidosis
If the lungs fail to eliminate CO2, hypercapnia and respiratory acidosis result, characterized by increased PaCO2 and decreased blood pH. Acute and chronic causes can be differentiated by examining arterial pH, PaCO2, and HCO3− values. In the early phase of respiratory acidosis, increased PaCO2 stimulates renal generation and secretion of H+. The kidneys continue to adapt to the increased pH through greater titratable acid excretion (e.g., ammonium) and HCO3− generation. Therefore, acute respiratory acidosis is characterized by an elevated PaCO2, acidemia, and a relatively normal HCO3−. In contrast, chronic respiratory acidosis is associated with an elevated HCO3− (often accompanied by a relatively normal pH) due to renal compensation.
Respiratory Alkalosis
Increased minute ventilation is the primary cause of respiratory alkalosis, characterized by decreased PaCO2 and increased pH. Patients with acute, uncompensated respiratory alkalosis have normal plasma HCO3−. In chronic respiratory alkalosis, renal compensation leads to decreased plasma HCO3−. The causes of respiratory alkalosis relate to abnormal respiratory drive from stimulants or toxins (e.g., salicylate, caffeine, nicotine, progesterone), central nervous system abnormalities (e.g., anxiety, stroke, increased intracranial pressure), pulmonary abnormalities (e.g., pulmonary embolism, pneumonia), mechanical hyperventilation, or systemic conditions such as liver failure and sepsis.
Mixed Acid–Base Disorders
It is not uncommon for a metabolic derangement to coexist with a respiratory derangement, particularly in intensive care patients. A general approach to the diagnosis of mixed acid–base disorders requires a step-wise approach that begins with a focused history and physical examination. An arterial blood gas and a concurrent serum chemistry panel (including Na+, K+, Cl−, and total CO2 concentrations) should also be obtained, and the use of an acid–base map may help differentiate simple from mixed disorders (Fig. 49-4).
FIGURE 49-4. Acid–base map. Plotting the PCO2 and H+ (from the arterial blood gas) against plasma HCO3− (from the serum chemistry panel) for a patient can identify simple acid–base disorders. When mixed disorders exist, values may fall outside the shaded areas. (From: DuBose TD Jr. In: Brenner BM, ed. Acid-Base Disorders, Brenner & Rector’s The Kidney. 7th ed. Philadelphia, PA: WB Saunders; 2004:938, with permission.)
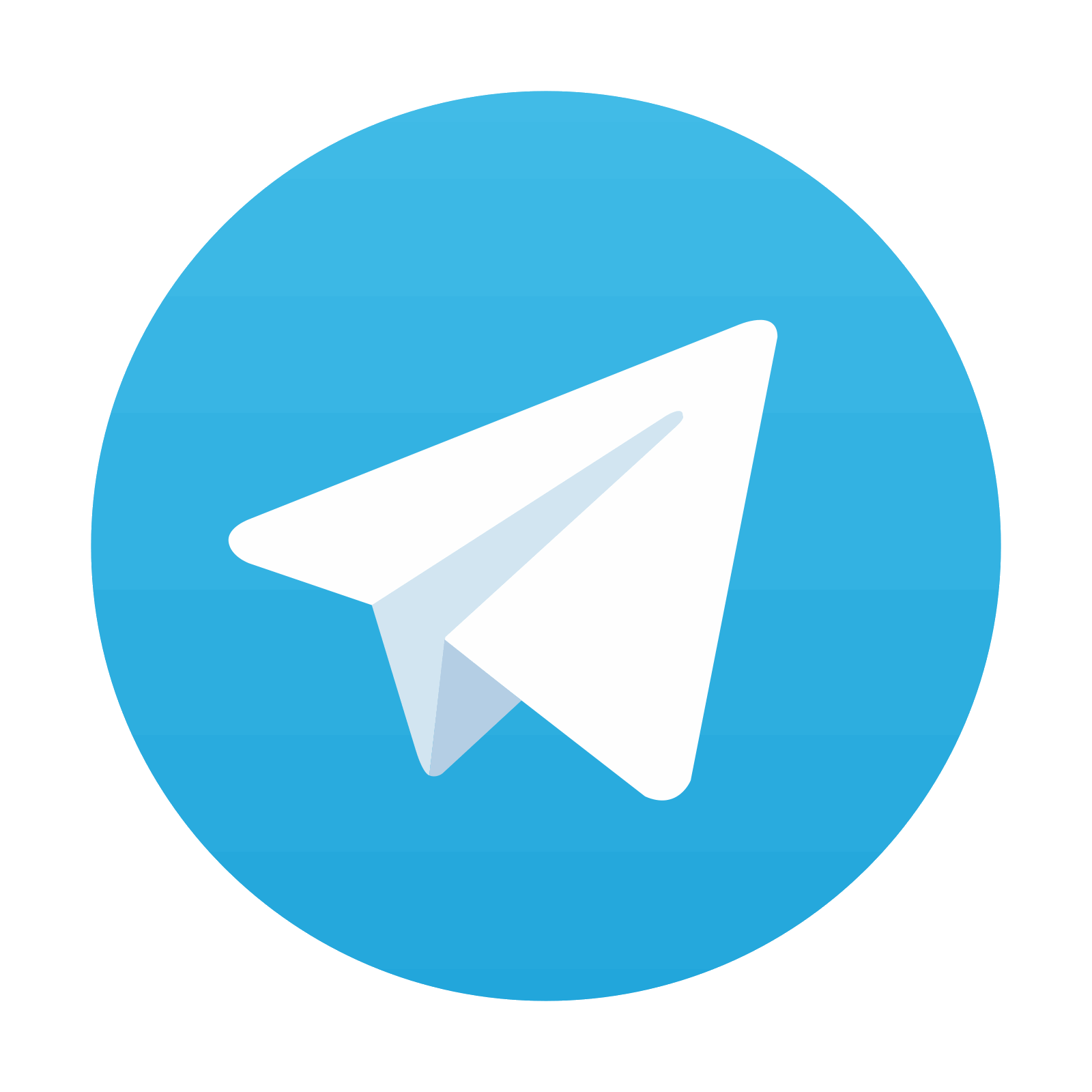
Stay updated, free articles. Join our Telegram channel
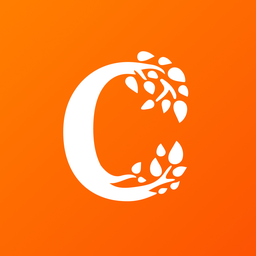
Full access? Get Clinical Tree
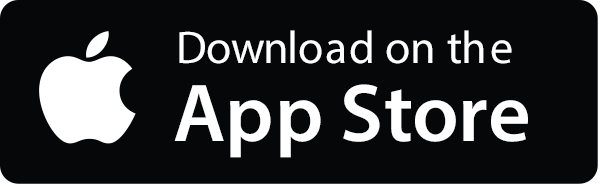
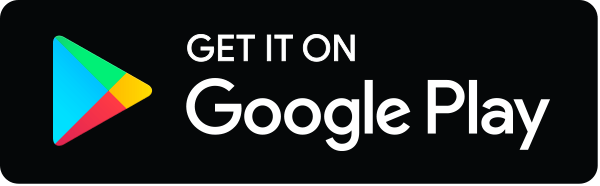