(99–55 BC)
The fundamental goal of nutritional support is to provide the daily nutrient and energy needs of each patient. This chapter will describe how to evaluate those needs in critically ill patients (1), and will try to do so without pretending that anyone knows how to support metabolism in patients who are critically ill.
DAILY ENERGY EXPENDITURE
Oxidation of Nutrient Fuels
Oxidative metabolism captures the energy stored in nutrient fuels (carbohydrates, lipids, and proteins) and uses this energy to sustain life. This process consumes oxygen, and generates carbon dioxide, water, and heat. The quantities involved in the oxidation of each type of nutrient fuel are shown in Table 47.1. The following points deserve mention.
1. The heat generated by the complete oxidation of a nutrient fuel is equivalent to the energy yield (in kcal/g) of that fuel.
2. Lipids have the highest energy yield (9.1 kcal/gram), while glucose has the lowest energy yield (3.7 kcal/gram).
The summed metabolism of all three nutrient fuels determines the whole-body O2 consumption (VO2), CO2 production (VCO2), and heat production for any given time period. The 24-hour heat production is equivalent to the daily energy expenditure (in kcal) for each patient. The daily energy expenditure determines how many calories to provide each day in nutritional support, and it can be calculated or measured.
Table 47.1 Oxidative Metabolism of Nutrient Fuels
Indirect Calorimetry
It is not possible to measure metabolic heat production in hospitalized patients, but if the whole-body O2 consumption (VO2) and CO2 production (VCO2) are known, the relationships in Table 47.1 can be used to determine the metabolic heat production. This is the principle of indirect calorimetry, which measures the resting energy expenditure (REE) using the following relationships (2):
(47.1)
Methodology
Indirect calorimetry is performed with “metabolic carts” that measure whole-body VO2 and VCO2 at the bedside by measuring the concentrations of O2 and CO2 in inhaled and exhaled gas (usually in intubated pa-tients). Steady-state measurements are obtained for 15–30 minutes to determine the REE (kcal/min), which is then multiplied by 1,440 (the number of minutes in 24 hours) to derive the daily energy expenditure (kcal/24 hr) (3). Clinical studies have shown that REE measurements obtained over 30 minutes and extrapolated to 24 hours are equivalent to REE measurements performed for 24 hours (4). The oxygen sensor in metabolic carts is not reliable at O2 concentrations above 60% (3), so indirect calorimetry can be unreliable when inhaled O2 concentrations are ≥60%.
Although indirect calorimetry is the most accurate method for determining daily energy requirements, it requires expensive equipment along with trained personnel, and is not universally available. As a result, daily energy requirements are usually estimated, as described next.
The Simple Way
There are more than 200 cumbersome equations available for estimating daily energy requirements (1), but none is considered more predictive than the following relationship:
(47.2)
This simple predictive relationship is remarkably accurate in most ICU patients (5) and is considered suitable for estimating daily energy requirements in the ICU (1). Actual body weight is used unless it is 25% higher than ideal body weight. When actual weight is more than 125% of ideal weight, the adjusted weight (wt) can be used, as determined by the following equation (6):
(47.3)
SUBSTRATE REQUIREMENTS
Nonprotein Calories
The daily energy requirement is provided by nonprotein calories from carbohydrates and lipids, while protein intake is used to maintain essential enzymatic and structural proteins.
Carbohydrates
Standard nutrition regimens use carbohydrates to provide about 70% of the nonprotein calories. The human body has limited carbohydrate stores (Table 47.2), and daily intake of carbohydrates is necessary to ensure proper functioning of the central nervous system, which relies heavily on glucose as a nutritive fuel. However, excessive carbohydrate intake promotes hyperglycemia, which has several deleterious effects, including impaired immune responsiveness in leukocytes (7).
Table 47.2 Endogenous Fuel Stores in Healthy Adults
Lipids
Standard nutrition regimens use lipids to provide approximately 30% of the daily energy needs. Dietary lipids have the highest energy yield of the three nutrient fuels (Table 47.1), and lipid stores in adipose tissues represent the major endogenous fuel source in healthy adults (Table 47.2).
Linoleic Acid
Dietary lipids are triglycerides, which are composed of a glycerol molecule linked to three fatty acids. The only dietary fatty acid that is considered essential (i.e., must be provided in the diet) is linoleic acid, a long chain, polyunsaturated fatty acid with 18 carbon atoms (8). A deficient intake of this essential fatty acid produces a clinical disorder characterized by a scaly dermopathy, cardiac dysfunction, and increased susceptibility to infections (8). This disorder is prevented by providing 0.5% of the dietary fatty acids as linoleic acid. Safflower oil is used as the source of linoleic acid in most nutritional support regimens.
Propofol
Propofol, an intravenous anesthetic agent that is popular for short-term sedation in the ICU, is mixed in a 10% lipid emulsion very similar to 10% Intralipid (Baxter Healthcare) that provides 1.1 kcal/mL. As a result, the calories provided by propofol infusions must be considered when calculating the nonprotein calories in a nutrition support regimen (1).
Protein Requirements
The daily protein requirement is dependent on the rate of protein catabolism. The normal daily protein intake is 0.8–1 grams/kg, but in ICU patients, the daily protein intake is higher at 1.2–1.6 grams/kg because of hypercatabolism (9).
Nitrogen Balance
The adequacy of protein intake can be evaluated with the nitrogen balance; i.e., the difference between intake and excretion of protein-derived nitrogen.
1. Nitrogen Excretion: Two-thirds of the nitrogen derived from protein breakdown is excreted in the urine (8), and about 85% of this nitrogen is contained in urea (the remainder is in ammonia and creatinine). Thus, the urinary urea nitrogen (UUN), measured in grams excreted in 24 hours, represents the bulk of nitrogen derived from protein breakdown. The remainder of the protein-derived nitrogen (usually about 4–6 grams/day) is excreted in the stool. Therefore, protein-derived nitrogen excretion can be expressed as follows:
(47.4)
If the UUN is greater than 30 g/24 h, 6 grams is more appropriate for the estimated non-urinary nitrogen losses (10). In the presence of diarrhea, non-urinary nitrogen losses cannot be estimated accurately, and nitrogen balance determinations are unreliable.
2. Nitrogen Intake: Protein is 16% nitrogen, so each gram of protein contains 1/6.25 grams of nitrogen. Therefore, protein-derived nitrogen intake is derived as follows:
(47.5)
3. Nitrogen Balance: The equations for nitrogen intake and nitrogen excretion are combined to determine the daily nitrogen balance.
(47.6)
The goal of nutritional support is a positive nitrogen balance of 4–6 grams.
FIGURE 47.1 Relationship between nitrogen balance and the daily intake of nonprotein calories relative to daily calorie requirements. Protein intake is constant. REE = resting energy expenditure.
Nitrogen Balance & Nonprotein Calories
The first step in achieving a positive nitrogen balance is to provide enough nonprotein calories to spare proteins from being degraded to provide energy. This is demonstrated in Figure 47.1. When the daily protein intake is constant, the nitrogen balance becomes positive only when the intake of nonprotein calories is sufficient to meet the daily energy needs (i.e., the REE). Therefore, increasing protein intake will not achieve a positive nitrogen balance unless the intake of nonprotein calories is adequate.
VITAMIN REQUIREMENTS
Thirteen vitamins are considered an essential part of the daily diet, and Table 47.3 shows the recommended daily dose and the maximum tolerable daily dose of these vitamins. The daily vitamin requirements have not been identified in critically ill patients (and probably vary with each patient) but they are likely to be higher than the recommended daily doses in Table 47.3. This is supported by reports of vitamin deficiencies in hospitalized patients who were receiving daily vitamin supplementation (11,12). Two vitamin deficiencies that deserve mention are described next.
Thiamine Deficiency
Thiamine (vitamin B1) plays an essential role in carbohydrate metabolism, where it serves as a coenzyme (thiamine pyrophosphate) for pyruvate dehydrogenase, the enzyme that allows pyruvate to enter mitochondria and undergo oxidative metabolism to generate high-energy ATP molecules (13). Thiamine deficiency can thus have an adverse effect on cellular energy production, particularly in the brain, which relies heavily on glucose metabolism.
Table 47.3 Dietary Allowances for Vitamins
Predisposing Factors
The prevalence of thiamine deficiency in ICU patients is not known, but the are several conditions in ICU patients that promote thiamine deficiency, including alcoholism, hypermetabolic states like trauma (14), increased urinary thiamine excretion by furosemide (15), and magnesium depletion (16). Furthermore, thiamine is degraded by sulfites (used as preservatives) in parenteral nutrition solutions (17), so thiamine-containing multivitamin preparations should not be mixed with parenteral nutrition solutions.
Clinical Manifestations
The consequences of thiamine deficiency include cardiomyopathy (wet beriberi), Wernicke’s encephalopathy (18), lactic acidosis (19), and a peripheral neuropathy (dry beriberi). Cardiomyopathies, encephalo-pathies, and lactic acidosis are common in ICU patients, and the possible contribution of thiamine deficiency to these conditions should not be overlooked.
Diagnosis
The laboratory evaluation of thiamine status is shown in Table 47.4. Plasma levels of thiamine can be useful in detecting thiamine depletion, but the most reliable measure of functional thiamine stores is the erythrocyte transketolase assay (21). This assay measures the activity of a thiamine pyrophosphate-dependent (transketolase) enzyme in the patient’s red blood cells in response to the addition of thiamine pyrophosphate (TPP). An increase in enzyme activity of greater than 25% after the addition of TPP indicates functional thiamine deficiency.
Table 47.4 Laboratory Evaluation of Thiamine Status
Vitamin E Deficiency
Vitamin E is the major lipid-soluble antioxidant in the body, and plays a major role in preventing damage from lipid peroxidation in cell membranes (22). The incidence of vitamin E deficiency in ICU patients is not known, but vitamin E deficiency is common during parenteral nutrition (23). The reperfusion injury that follows aortic cross-clamping is associated with reduced blood levels of vitamin E, and pre-treatment with vitamin E ameliorates this reperfusion injury (24). Considering that oxidant stress plays an important role in the pathogenesis of inflammatory-mediated organ injury (25), attention to the status of vitamin E in critically ill patients seems warranted. The normal plasma concentration of vitamin E is 11.6–30.8 µmol/L (0.5–1.6 mg/dL) (26).
ESSENTIAL TRACE ELEMENTS
Daily Requirements
A trace element is a substance that is present in the body in amounts less than 50 µg per gram of body tissue (27). Seven trace elements are considered essential in humans (i.e., are associated with deficiency syndromes), and these are listed in Table 47.5, along with the recommended and maximum daily intake for each element. As mentioned for vitamins, the essential trace element requirements are not known in critically ill patients, and are probably higher than normal. The following trace elements deserve mention because of their relevance to oxidant cell injury.
Table 47.5 Dietary Allowances for Essential Trace Elements
Iron
One of the interesting features of iron in the human body is how little is allowed to remain as free, unbound iron. The normal adult has approximately 4.5 grams of iron, yet there is virtually no free iron in plasma (28). Most of the iron is bound to hemoglobin, and the remainder is bound to ferritin in tissues and transferrin in plasma. Furthermore, the transferrin in plasma is only approximately 30% saturated with iron, so any increase in plasma iron will be quickly bound by transferrin, thus preventing any rise in plasma free iron.
Iron and Oxidant Injury
One reason for the paucity of free iron is the ability of free iron to promote oxidant-induced cell injury (28,29). Iron in the reduced state (Fe-II) promotes the formation of hydroxyl radicals (see Figure 22.6), and hydroxyl radicals are considered the most reactive oxidants known in biochemistry. In this context, the ability to bind and sequester iron has been called the major antioxidant function of blood (29). This might explain why hypoferremia is a common occurrence in patients who have conditions associated with hypermetabolism (30) (because this would limit the destructive effects of hypermetabolism).
In light of this description of iron, a reduced serum iron level in a critically ill patient should not prompt iron replacement therapy unless there is evidence of total-body iron deficiency. This latter condition can be detected with a plasma ferritin level; i.e., iron deficiency is likely if the plasma ferritin is below 18 µg/L, and is unlikely if the plasma ferritin is above 100 µg/L (31).
Selenium
Selenium is an endogenous antioxidant by virtue of its role as a co-factor for glutathione peroxidase (see Figure 22.7). The recommended daily re-quirement for selenium is 55 µg in healthy adults (32), but selenium utilization is increased in acute illness (33), so daily requirements are likely to be higher in critically ill patients. A recent review of studies evaluating selenium in patients with severe sepsis has shown that low plasma levels of selenium are common in severe sepsis, and selenium supplementation in severe sepsis is associated with a lower mortality rate (34). In light of this study, attention to monitoring serum selenium levels in severe sepsis, as well as other conditions associated with systemic inflammation, seems warranted. The normal plasma selenium concentration is 89–113 µg/L (35).
A FINAL WORD
The Problem with Nutrition Support in Critically Ill Patients
Before leaving this chapter, it is important to point out a fundamental problem with promoting nutrient intake in critically ill patients (36). The problem is the difference in mechanisms for the malnutrition associated with critical illness and the malnutrition associated with starvation; i.e., the malnutrition from starvation is due to depletion of essential nutrients, while the malnutrition associated with critical illnesses is the result of abnormal nutrient processing. Because malnutrition in critically ill patients is caused by metabolic derangements, providing nutrients will not correct the malnutrition until the metabolic derangements resolve.
FIGURE 47.2 Influence of dextrose infusion on arterial lactate levels during abdominal aortic surgery. Each point represents the mean lactate level for 10 patients receiving Ringer’s solution (closed squares) and 10 patients receiving 5% dextrose solution (open squares). Total volume infused is equivalent with both fluids. Data from Reference 38.
An example of abnormal nutrient processing in acute illness is illustrated by the fate of a glucose load; i.e., less than 5% of glucose is metabolized to lactate in healthy subjects, while as much as 85% of a glucose load can be recovered as lactate in acutely ill patients (37). This is demonstrated in Figure 47.2 (38). In this case, patients undergoing abdominal aneurysm surgery were given intraoperative fluid therapy with either Ringer’s solutions or 5% dextrose solutions. In the patients who received dextrose (an average of 200 grams), the blood lactate level increased by more than 3 mmol/L, whereas the blood lactate level increased <1 mmol/L in the patients who received dextrose-free fluids. This demonstrates that nutrient intake can have very different consequences in critically ill patients, and the consequences can be toxic (e.g., the accumulation of an organic acid). It seems Lucretius had the right idea, over 2,000 years ago.
REFERENCES
Clinical Practice Guideline
1. McClave SA, Martindale RG, Vanek VW, et al. Guidelines for the provision and assessment of nutrition support therapy in the adult critically ill patient: Society of Critical Care Medicine and American Society for Parenteral and Enteral Nutrition. J Parent Ent Nutr 2009; 33:277–316.
Daily Energy Expenditure
2. Bursztein S, Saphar P, Singer P, et al. A mathematical analysis of indirect calorimetry measurements in acutely ill patients. Am J Clin Nutr 1989; 50:227–230.
3. Lev S, Cohen J, Singer P. Indirect calorimetry measurements in the ventilated critically ill patient: facts and controversies – the heat is on. Crit Care Clin 2010; 26:e1–e9.
4. Smyrnios NA, Curley FJ, Shaker KG. Accuracy of 30-minute indirect calorimetry studies in predicting 24-hour energy expenditure in mechanically ventilated critically ill patients. J Parenter Enteral Nutr 1997; 21:168–174.
5. Paauw JD, McCamish MA, Dean RE, et al. Assessment of caloric needs in stressed patients. J Am Coll Nutr 1984; 3:51–59.
6. Krenitsky J. Adjusted body weight, pro: Evidence to support the use of adjusted body weight in calculating calorie requirements. Nutr Clin Pract 2005; 20:468–473.
Substrate Requirements
7. Marik PE, Preiser J-C. Toward understanding tight glycemic control in the ICU. Chest 2010; 137:544–551.
8. Jones PJH, Kubow S. Lipids, Sterols, and Their Metabolites. In: Shils ME, et al., eds. Modern nutrition in health and disease. 10th ed. Philadelphia, PA: Lippincott, Williams & Wilkins, 2006; 92–121.
9. Matthews DE. Proteins and Amino Acids. In: Shils ME, et al., eds. Modern nutrition in health and disease. 10th ed. Philadelphia, PA: Lippincott, Will-iams & Wilkins, 2006; 23–61.
10. Velasco N, Long CL, Otto DA, et al. Comparison of three methods for the estimation of total nitrogen losses in hospitalized patients. J Parenter Enteral Nutr 1990; 14:517–522.
Vitamin Requirements
11. Dempsey DT, Mullen JL, Rombeau JL, et al. Treatment effects of parenteral vitamins in total parenteral nutrition patients. J Parenter Enteral Nutr 1987; 11:229–237.
12. Beard ME, Hatipov CS, Hamer JW. Acute onset of folate deficiency in patients under intensive care. Crit Care Med 1980; 8:500–503.
13. Butterworth RF. Thiamine. In: Shils ME, et al., eds. Modern nutrition in health and disease. 10th ed. Philadelphia, PA: Lippincott, Williams & Wilkins, 2006; 426–433.
14. McConachie I, Haskew A. Thiamine status after major trauma. Intensive Care Med 1988; 14:628–631.
15. Seligmann H, Halkin H, Rauchfleisch S, et al. Thiamine deficiency in patients with congestive heart failure receiving long-term furosemide therapy: a pilot study. Am J Med 1991; 91:151–155.
16. Dyckner T, Ek B, Nyhlin H, et al. Aggravation of thiamine deficiency by magnesium depletion. A case report. Acta Med Scand 1985; 218:129–131.
17. Scheiner JM, Araujo MM, DeRitter E. Thiamine destruction by sodium bisulfite in infusion solutions. Am J Hosp Pharm 1981; 38:1911–1916.
18. Tan GH, Farnell GF, Hensrud DD, et al. Acute Wernicke’s encephalopathy attributable to pure dietary thiamine deficiency. Mayo Clin Proc 1994; 69:849–850.
19. Oriot D, Wood C, Gottesman R, et al. Severe lactic acidosis related to acute thiamine deficiency. J Parenter Enteral Nutr 1991; 15:105–109.
20. Koike H, Misu K, Hattori N, et al. Postgastrectomy polyneuropathy with thiamine deficiency. J Neurol Neurosurg Psychiatry 2001; 71:357–362.
21. Boni L, Kieckens L, Hendrikx A. An evaluation of a modified erythrocyte transketolase assay for assessing thiamine nutritional adequacy. J Nutr Sci Vitaminol (Tokyo) 1980; 26:507–514.
22. Burton GW, Ingold KU. Vitamin E as an in vitro and in vivo antioxidant. Ann NY Acad Sci 1989; 570:7–22.
23. Vandewoude MG, Vandewoude MFJ, De Leeuw IH. Vitamin E status in patients on parenteral nutrition receiving intralipid. J Parenter Enter Nutr 1986; 10:303–305.
24. Novelli GP, Adembri C, Gandini E, et al. Vitamin E protects human skeletal muscle from damage during surgical ischemia-reperfusion injury. Am J Surg 1996; 172:206–209.
25. Anderson BO, Brown JM, Harken AH. Mechanisms of neutrophil-mediated tissue injury. J Surg Res 1991; 51:170–179.
26. Meydani M. Vitamin E. Lancet 1995; 345:170–175.
Essential Trace Elements
27. Fleming CR. Trace element metabolism in adult patients requiring total parenteral nutrition. Am J Clin Nutr 1989; 49:573–579.
28. Halliwell B, Gutteridge JM. Role of free radicals and catalytic metal ions in human disease: an overview. Methods Enzymol 1990; 186:1–85.
29. Herbert V, Shaw S, Jayatilleke E, et al. Most free-radical injury is iron-related: it is promoted by iron, hemin, holoferritin and vitamin C, and inhibited by desferoxamine and apoferritin. Stem Cells 1994; 12:289–303.
30. Shanbhogue LK, Paterson N. Effect of sepsis and surgery on trace minerals. J Parenter Enteral Nutr 1990; 14:287–289.
31. Guyatt GH, Patterson C, Ali M, et al. Diagnosis of iron-deficiency anemia in the elderly. Am J Med 1990; 88:205–209.
32. Food and Nutrition Board, Institute of Medicine. Recommended dietary allowances and adequate intakes of trace elements. Available at the Food and Nutrition website (http://fnic.nal.usda.gov), accessed July, 2013.
33. Hawker FH, Stewart PM, Snitch PJ. Effects of acute illness on selenium homeostasis. Crit Care Med 1990; 18:442–446.
34. Alhazzani W, Jacobi J, Sindi A, et al. The effect of selenium therapy on mortality in patients with sepsis syndrome. Crit Care Med 2013; 41:1555–1564.
35. Geoghegan M, McAuley D, Eaton S, et al. Selenium in critical illness. Curr Opin Crit Care 2006; 12:136–141.
A Final Word
36. Marino PL, Finnegan MJ. Nutrition support is not beneficial and can be harmful in critically ill patients. Crit Care Clin 1996; 12:667–676.
37. Gunther B, Jauch KW, Hartl W, et al. Low-dose glucose infusion in patients who have undergone surgery. Possible cause of a muscular energy deficit. Arch Surg 1987; 122:765–771.
38. Degoute CS, Ray MJ, Manchon M, et al. Intraoperative glucose infusion and blood lactate: endocrine and metabolic relationships during abdominal aortic surgery. Anesthesiology 1989; 71:355–361.
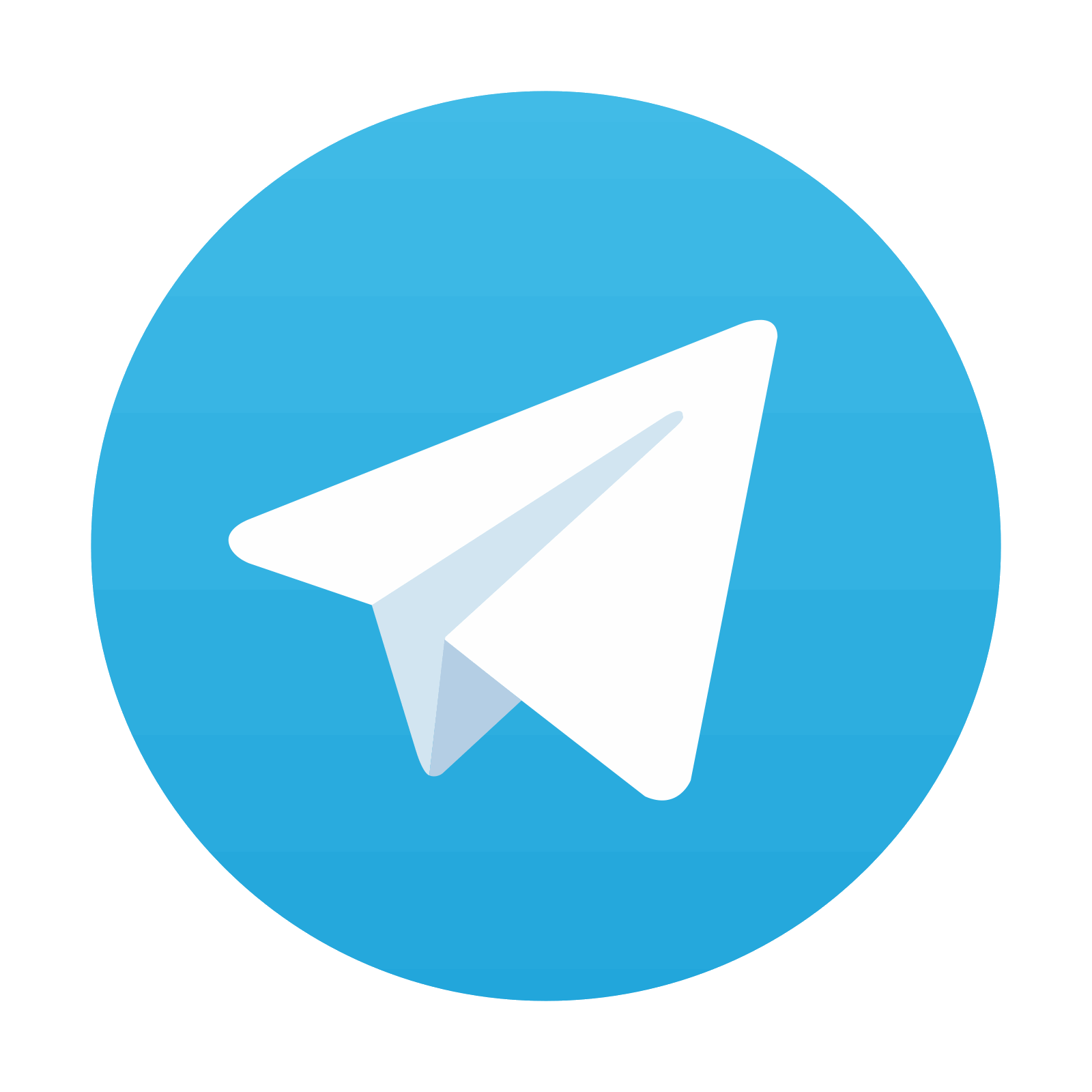
Stay updated, free articles. Join our Telegram channel
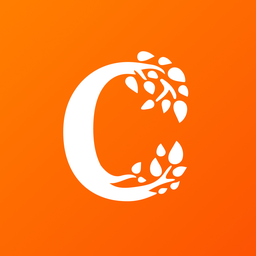
Full access? Get Clinical Tree
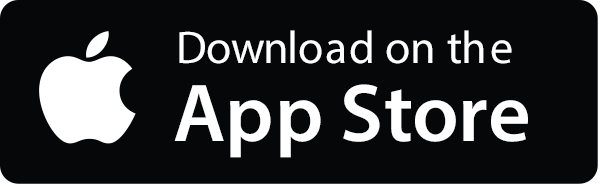
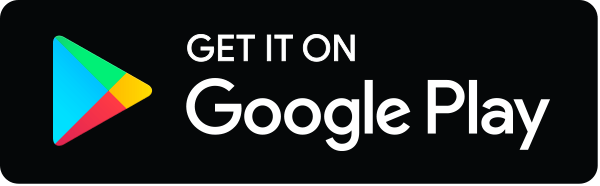