Anatomically, blood flow to the normal brain is supplied by the two carotid arteries and vertebral arteries. Collateral circulation is provided via the Circle of Willis.
Physiologically, blood flow to the brain is tightly regulated. The homeostatic mechanisms include flow–metabolism coupling, pressure autoregulation, and CO2 reactivity.
These homeostatic mechanisms are affected by diseases as well as anesthetic drugs and techniques.
Multiple monitoring modalities are available to monitor brain function, perfusion, and oxygenation/metabolism. These include the electroencephalogram, somatosensory evoked potentials, motor evoked potentials, electromyogram, intracranial pressure, transcranial Doppler ultrasonography, brain tissue oxygenation, and jugular venous oximetry. Although most are applicable for monitoring in the neurointensive care unit, many are useful in the operating room to increase patient safety and improve outcome.
Definitive cerebral protective therapy remains elusive, but many techniques have been investigated and some are frequently used in the operating room on theoretical grounds. These include the use of hypothermia, control of blood glucose, and maintenance of adequate perfusion. The anemia threshold for blood transfusion remains controversial.
Anesthetic management of the patient with neurologic disease mandates a thorough preoperative assessment as there are often multisystem manifestations.
Anesthetic techniques may influence brain relaxation conditions. In general, intravenous agents cause more cerebral vasoconstriction than inhalation agents. There are no outcome studies demonstrating the superiority of any particular anesthetic agent. The use of intraoperative monitoring of evoked potentials impacts the choice of anesthetic technique.
Movement of water into the brain is primarily determined by the osmotic gradient, which in turn is determined by serum osmolarity. Outcome studies do not provide guidance regarding the choice of crystalloids versus colloids. In patients with brain trauma the use of albumin for resuscitation is associated with increased mortality.
Common neurosurgical procedures requiring special understanding and expertise include tumor excision, transsphenoidal or transcranial removal of pituitary lesions, extirpation of arteriovenous malformation, repair or clipping of aneurysms, carotid endarterectomy, and craniotomy for traumatic brain lesions including epidural and subdural hematomas.
Multimedia
Intracranial Compliance
Cerebral Blood Flow
Neurosurgery Tumor Excision
Mayfield Clamp
NEUROANATOMY
A basic knowledge of neuroanatomy is essential for all anesthesiologists, particularly those caring for patients with disease of the central nervous system (CNS). Components of the CNS, the brain and spinal cord, are protected by the bony structures that surround them. Yet by virtue of their protective nature, these structures are nondistensible. The intracranial volume is fixed, thereby providing little room for anything other than the brain, cerebrospinal fluid (CSF), and blood contained in the cerebral vasculature. Even the space in the spinal column, although not as restrictive as the cranium, is quickly exhausted by a space-occupying lesion such as an expanding hematoma or abscess. It is in the context of the restrictive nature of the cranium and vertebral column in which the CNS is housed that all interventions must be considered.
Both the brain and spinal cord have unique blood supply. The carotid artery in the neck bifurcates into the external and internal carotid arteries at the level of the third cervical vertebra, sending the internal branch through the base of the skull, giving seven branches including the ophthalmic artery, and ultimately bifurcating into the anterior and middle cerebral arteries. These vessels define the anterior cerebral circulation. The posterior circulation results from the vertebral arteries, which ascend in the posterior aspect of the neck through foramina in the cervical vertebral bodies before exiting, coursing around the brainstem, and joining the contralateral vessel to form the basilar artery. The basilar artery ascends along the brainstem before dividing into the posterior cerebral arteries. The anterior and posterior circulations anastomose through the posterior communicating arteries to provide collateral flow; collateral circulation can also occur through the anterior communicating artery connecting the bilateral anterior cerebral arteries. This system of collateralization, named the circle of Willis (Fig. 36-1), was described by Thomas Willis (1621–1675) with the recognition of its purpose “ … that there may be a manifold way, and that more certain, for the blood about to go into divers Regions of the Brain.”
FIGURE 36-1. The circle of Willis, and other blood supply to the brain and spinal cord.
The spinal column is the bony structure made up of the 7 cervical, 12 thoracic, 5 lumbar vertebrae, 5 fused sacral and 3 to 5 fused coccygeal vertebrae. It is about 70 cm long in the adult male with cervical and lumbar regions which convex forward and thoracic and sacral regions that are concave. The spinal cord exits the skull through the foramen magnum and enters the canal formed by the vertebral bodies. In the adult, the cord typically ends at the lower aspect of the first lumbar vertebral body. It is around 45 cm long in men and 43 cm long in women.
Blood supply to the cord is provided by several sources. The anterior spinal artery, which arises from the vertebral arteries, supplies the anterior two-thirds of the spinal cord. This vessel runs the length of the cord, receiving contributions from radicular arteries via intercostal vessels. The artery of Adamkiewicz is the most important radicular vessel, typically joining the anterior spinal artery in the lower thoracic region and providing blood to the thoracolumbar cord. The posterior third of the cord is supplied by two posterior spinal arteries, which arise from the vertebral arteries and also receive contributions from radicular arteries (Fig. 36-2).
FIGURE 36-2. Blood supply to the spinal cord. Both the single anterior spinal artery and the paired posterior spinal artery arise from the vertebral arteries. The radicular arteries and particularly the artery of Adamkiewicz are important contributors. The anterior spinal artery supplies the anterior two-thirds of the spinal cord, with the posterior spinal artery supplying the rest. (vert., vertebral; art., artery; ant., anterior)
NEUROPHYSIOLOGY
Cerebral metabolic rate is directly related to the number of stimulated neurons and rate of depolarization. Therefore, any activity or stimulation raises the metabolic rate. Cerebral blood flow (CBF) is tightly coupled to metabolism, on a regional as well on a global level. As an example, while visual stimulation may raise blood flow to the occipital cortex, mild hyperthermia, which raises global cerebral metabolic rate, increases flow to the entire brain.
The CSF occupies the subarachnoid space, providing a protective layer of fluid between the CNS and the tissue that surrounds it. CSF is produced by the choroid plexus in the ventricles at about 0.3 mL/min. CSF circulation follows the path from the lateral ventricles into the third ventricle via the interventricular foramina (foramina of Monro). It subsequently transits through the cerebral aqueduct of Sylvius into the fourth ventricle, and then into the space around the brain via the foramina of Magendie (midline posteriorly) and Luschka (laterally). It bathes both the spinal cord and the brain. Absorption into the dural venous sinuses occurs through the arachnoid granulations. Although CSF volume is approximately 150 mL, more than three times this amount is produced in a 24-hour period. This continuous flow of CSF from the source to sink allows it to participate in many functions in addition to cushioning the brain. It maintains a milieu in which the brain can function by regulating pH and electrolytes, carrying away waste products, and delivering nutrients.1,2
Intracranial pressure (ICP) is low except in pathologic states. The Monro–Kellie doctrine states that in the setting of a nondistensible cranial vault, the volume of blood, CSF, and brain tissue must be in equilibrium. An increase in one of these three elements, or the addition of a space-occupying lesion, can be accommodated initially through displacement of CSF into the thecal sac, but only to a small extent. A further increase, as with significant cerebral edema or accumulation of an extradural hematoma, will quickly lead to a marked increase in ICP due to limited intracranial compliance (Fig. 36-3).
FIGURE 36-3. Intracranial compliance (elastance) curve. The brain has minimal compensatory capacity, and any increase in mass from hematoma or brain swelling will result in an inordinate increase in intracranial pressure.
As mentioned earlier, blood flow to the brain is tightly coupled to cerebral metabolism. As such, many factors affect CBF because of their effect on metabolism. Stimulation, arousal, nociception, and mild hyperthermia elevate metabolism and flow, while sedative–hypnotic agents and hypothermia decrease both metabolism and flow
. A number of other factors govern CBF directly without changing metabolism. A potent determinant of CBF is the arterial CO2 tension (PaCO2). Within physiologic range, CBF has an approximately linear relationship with PaCO2. CBF changes by approximately 3% of baseline for each 1 mm Hg change in PaCO2 (Fig. 36-4). As CBF changes, so does cerebral blood volume (CBV), which is why hyperventilation can be used for short periods of time to relax the brain or decrease the ICP. However, this effect is thought to be short-lived. CSF pH normalizes over time, and vessel caliber returns to baseline. The exact duration of hypocapnic vasoconstriction is uncertain; a period of minutes to hours has been found in different patient populations.3 Because the decrease in CBF occurs without a change in cerebral metabolic rate, the risk of ischemia is a theoretical concern. However, the significance of this concern is uncertain. We have no evidence of harm of moderate hyperventilation to the normal brain under general anesthesia. Early hyperventilation in traumatic brain injury (TBI) is associated with poor outcome, and the consequence of hyperventilation in TBI after the initial 24 hours is uncertain.4–6
FIGURE 36-4. Cerebrovascular response to change in PaCO2 partial pressure. The change is linear between PaCO2 of 25 and 65 mm Hg.
In contrast to CO2, O2 has little effect on CBF except at abnormally low levels (Fig. 36-5). When PaO2 falls below 50 mm Hg, CBF begins to increase sharply. A teleologic explanation for this phenomenon is that CBF needs to increase only when the O2 content of the blood begins to decrease significantly.
FIGURE 36-5. Cerebrovascular response to change in PaO2 partial pressure. The response of cerebral blood flow to change in PaO2 is flat until PaO2 falls below 50 mm Hg.
CBF remains approximately constant despite modest swings in arterial blood pressure. The mechanism by which CBF is maintained, originally described by Lassen,7 is called autoregulation of CBF, or at times, pressure autoregulation of CBF. As cerebral perfusion pressure (CPP), defined as the difference of mean arterial pressure (MAP) and ICP, changes, cerebrovascular resistance adjusts to maintain stable flow. The resistance is varied at the arteriolar level. The range of CPP over which autoregulation is maintained is termed the autoregulatory plateau. Although this range is frequently quoted as a MAP range of 60 to 150 mm Hg, there is significant variability between individuals, and these numbers are only approximate. At the low end of the plateau, cerebrovascular resistance is at a minimum, and any further decrease in CPP will compromise CBF. At the high end of the plateau, cerebrovascular resistance is at a maximum, and any further increase in CPP will result in hyperemia (Fig. 36-6). Various mechanisms have been proposed to account for autoregulation, including myogenic, neurogenic, and local metabolic mediators. However, the exact mechanism remains undefined.
FIGURE 36-6. Cerebral autoregulation. It is generally accepted that cerebral blood flow is maintained constant between 60 and 160 mm Hg. However, these are average values, and there is considerable variation in both the lower and the upper limit of cerebral autoregulation among normal individuals.
There is interaction between CO2 reactivity and pressure autoregulation, although the molecular mechanism is likely different for these two homeostatic processes. When blood pressure is low, CO2 reactivity is reduced. In contrast, under hypercapnic conditions, autoregulatory capacity is lost because of the concurrent vasodilation.
Other factors affect CBF as well. Anemia increases CBF via higher cardiac output, CPP and lower blood viscosity, as well as induced cerebral vasodilatation.8–10 Proposed mechanisms underlying this dilatation may include upregulation of nitric oxide (NO) production, sympathetic β2-receptor stimulation and upregulation of vascular endothelial growth factor, hypoxia-inducible factor 1α, and erythropoietin that contributes to vasodilatation and maintenance of CBF. Although it seems likely that these mediators are neuroprotective, it remains possible that they could also have harmful pathophysiologic effects.11–13
Anesthetic Influences
Anesthetic agents have variable influence on CBF and metabolism, CO2 reactivity, and autoregulation.14 Inhalation anesthetics tend to cause vasodilation in a dose-related manner, but do not per se uncouple flow and metabolism. Thus the vasodilatory influence is opposed by metabolism-mediated decrease in flow. The resultant effect is that during low doses of inhalation anesthesia, CBF is either unchanged or slightly increased. Higher doses result in dominance of the vasodilatory effect and an increase in CBF. Compared to other inhaled agents, sevoflurane in clinically relevant doses does not increase CBF, although propofol at comparable doses results in more profound cerebral vasoconstriction, and sevoflurane does not appear to impair cerebrovascular autoregulation.15,16 Furthermore, sevoflurane anesthesia is associated with profound regional and global reduction in cerebral metabolic rate.17 Intravenous agents including thiopental and propofol cause vasoconstriction coupled with a reduction in metabolism.18 Ketamine, on the other hand, increases flow and metabolism.19 CO2 reactivity is a robust mechanism and is preserved under all anesthetic conditions.
Dexmedetomidine is a pure α2 agonist that is increasingly utilized to provide a state of “conscious sedation” associated with minimal respiratory depression and some analgesia. It may create an ideal state to facilitate procedures such as awake craniotomy, carotid endarterectomy (CEA) under regional anesthesia, carotid angioplasty and stenting, and other neurointerventional procedures. It appears to preserve flow–metabolism coupling in healthy volunteers,20 although its effects on the injured brain still need to be determined. It has been reported to have little effect on static cerebrovascular autoregulation (sCA) in healthy volunteers (unpublished observation), but it may weaken dynamic cerebrovascular autoregulation (dCA) and delay restoration of CBF velocity to normal with reduction in blood pressure.21
PATHOPHYSIOLOGY
The homeostatic mechanisms that ensure protection of the brain and spinal cord, removal of waste, and delivery of adequate O2 and substrate to the tissue can be interrupted through a multitude of mechanisms. Traumatic insults may result in contusion with subsequent edema formation, direct injury from depressed skull fractures or spine fractures, diffuse injury to neurons from rapid deceleration, and disruption of the vasculature, resulting in ischemia or hemorrhage. All of these insults may ultimately compromise CNS perfusion.
Mass lesions, such as tumors, may compress adjacent structures, raise ICP, and obstruct normal flow of CSF. Hemorrhage may be spontaneous or traumatic. Depending on its location, this may cause a mass effect, impair CSF circulation, or, in the case of subarachnoid blood, breakdown of the blood may lead to further ischemic injury by causing cerebral vasospasm.
Hydrocephalus is caused by an imbalance between CSF production and removal. It frequently results in the elevation of ICP. Hydrocephalus is commonly divided into two categories: Communicating hydrocephalus and obstructive hydrocephalus. The former is characterized by a failure to absorb CSF, typically because of dysfunctional arachnoid granulations. The latter may be caused by any direct obstruction or extrinsic compression of a passageway through which CSF must pass, such as the cerebral aqueduct. This obstruction, for example, may result from a clot within the space or from a tumor adjacent to it. Depending on the circumstances, hydrocephalus can have a subtle or dramatic presentation. For example, acute hydrocephalus following an intraventricular hemorrhage may result in a rapidly progressive obtundation that improves dramatically with external ventricular drainage. In contrast, normal pressure hydrocephalus may evolve over years, resulting in barely perceptible changes in cognition and gait.
MONITORING
Anesthesia for neurosurgery and spine surgery requires the standard American Society of Anesthesiologists monitoring for physiologic parameters. However, the risk imposed to the CNS by these surgical procedures may warrant more extensive monitoring. For many procedures, adequate oxygenation, ventilation, and systemic blood pressure do not ensure the well being of the brain and spinal cord. Instead, the integrity of the CNS needs to be evaluated intraoperatively with monitors that specifically detect CNS function, perfusion, or metabolism. At times, the monitoring modalities can be combined to provide greater information regarding the well being of the CNS.
Central Nervous System Function
Electroencephalogram
The electroencephalogram (EEG) is the quintessential cerebral function monitor. It records the electrical activity generated by the depolarization of cortical neurons that can be detected by surface electrodes placed on the scalp. Typically the activity is measured between two points on the scalp (bipolar), as there is no electrically neutral place from which to reference the signal. Other sources of electrical activity, such as that from the heart and muscles, must be filtered from the signal, otherwise they would overwhelm the small voltages generated by the cortical activity. Common-mode rejection, that is, rejection of signals common to both electrodes, allows interference from cardiac and muscle activity to be minimized.
The EEG can be used for intraoperative monitoring (IOM) and diagnosis. Monitoring provides information regarding functional assessment of the brain, the occurrence of ischemia, seizure activity, burst-suppression pattern, and potentially, depth of anesthesia. Several standardized systems of electrode placement have been developed to facilitate reliable and consistent EEG monitoring, the most common of which is the International 10–20 System. In brief, artificial meridians are generated on the scalp running front to back and side to side, where the 10 to 20 refers to the percentage of the distance across the scalp, either from the tragus to tragus or the nasion to inion, that defines the meridian (Fig. 36-7). Electrodes can be placed at the intersection of each meridian. Each such intersection or point is given a name—either a combination of letters and a number or two letters, where the final letter is Z. The letters are F for frontal, C for central, P for parietal, T for temporal, O for occipital, A for auricular, and Fp for frontal pole. A letter followed by an odd number is a point on the left hemisphere, while a letter followed by an even number is a point on the right hemisphere. Two letters, with the second letter a Z, indicate a point along the midline.
FIGURE 36-7. The international 10–20 system for electroencephalogram electrode montage. The odd numbers denote the left (L) hemisphere whereas the even numbers represent the right (R) hemisphere. See text for details.
Although sophisticated EEG monitoring for epilepsy evaluation may require recording of multiple channels, providing information on the activity between numerous points, EEG monitoring during anesthesia frequently uses a broad montage with fewer channels (two or four) to evaluate hemispheric activity. Once the signal is recorded, it can be evaluated in several ways. Viewing raw EEG may be appropriate at times, but subtle changes are difficult to detect, particularly for the infrequent user. However, the EEG can be processed to yield readily interpretable information. A common method used is frequency domain analysis. Using Fourier analysis, the apparent random activity of raw EEG can be broken down into a series of wave frequencies, the summation of which gives the overall EEG pattern. The range of frequencies seen in EEG is described in Table 36-1. The power (amplitude squared) at each frequency can then be plotted as a spectral array, whereby the effect of various influences such as anesthetic agents or an ischemic insult can be detected by how they modify the spectral analysis. A common parameter to include in the analysis of EEG is the spectral edge frequency, which is the frequency below which 95% of the power resides.
TABLE 36-1. ELECTROENCEPHALOGRAM FREQUENCIES
A progressive reduction in CBF will produce a reliable pattern change in the EEG, consisting of a loss of high-frequency activity, a loss of power, and the eventual progression to EEG silence. The monitor is therefore useful when surgical procedures jeopardize perfusion to the brain, such as when the carotid artery is cross-clamped during CEA. The EEG is particularly useful in this setting because the spectral analysis on the at-risk side can be compared in real time with the unaffected side, thus facilitating detection of ischemia by the resultant asymmetry of the spectral edge frequency.
However, the changes in the EEG spectrum seen with ischemia can occur as a result of other influences. Intravenous anesthetic agents such as propofol and thiopental, as well as inhaled agents such as isoflurane, will cause a similar decrease in the spectral edge frequency, with eventual progression to a drug-induced isoelectric EEG in a dose-related manner. During certain surgical procedures, such as extracranial-to-intracranial arterial bypass procedures, maximal suppression of cerebral metabolic rate is desirable to protect the brain during an ischemic insult. Under such circumstances, the anesthetic agent can be titrated against the EEG until the desired effect is achieved. Typically, instead of an isoelectric EEG, the goal is a state called burst suppression. In this state, periods of isoelectric EEG are punctuated by “bursts” of EEG activity. When burst suppression is the goal, a suppression ratio can be calculated as the percentage of an epoch in which the patient’s EEG is isoelectric. The suppression ratio allows one to achieve near-complete suppression (>90%) of EEG activity, while remaining certain that regular EEG activity will return in a short while with cessation of administration of the drug. In contrast, when complete isoelectric EEG is achieved, time to arousal becomes unpredictable. Other settings in which EEG monitoring and burst suppression may be useful are listed in Table 36-2.
Using a proprietary algorithm based on probabilistic analysis, a computer-processed EEG has been used to derive a dimensionless number to monitor the degree of hypnosis or the “depth of anesthesia.” The most commonly used monitor is the Bispectral index (BIS) where a number between 40 and 60 is considered optimal for the prevention of intraoperative awareness. However, a recent study failed to demonstrate the superiority of a BIS protocol over a protocol based on end-tidal anesthetic agent concentration monitoring in the prevention of intraoperative awareness.22
Evoked Potential Monitoring
Although EEG is a cerebral function monitor that detects spontaneous activity, evoked potential (EP) modalities detect signals that are the result of specific stimuli applied to the patient. These include somatosensory evoked potential (SSEP), brainstem auditory evoked potential (BAEP), visual evoked potential (VEP), and motor evoked potential (MEP).
The proposed benefit of EP monitoring is to identify the deterioration of neuronal function, thus enabling the opportunity to correct offending factors before becoming irreversible. Such factors include positioning of the patient (e.g., neck position, shoulder position), hypotension, hypothermia, and surgical intervention. In elective spinal surgery without EP monitoring, iatrogenic neurologic injuries have been estimated to be 0.46% for anterior cervical discectomy, 0.23% to 3.2% with scoliosis correction, and between 23.8% and 65.4% with intramedullary spinal cord tumor resection. A recent systematic review indicated that, although there is a high level of evidence that multimodal neurophysiologic monitoring is sensitive and specific for detecting intraoperative neurologic injury during spine surgery, there is very little evidence that an intraoperative response to a neuromonitoring alert reduces the rate of perioperative neurologic deterioration. Consequently there is a low level of evidence that it reduces the rate of new or worsening perioperative neurologic deficits.23,24
Somatosensory Evoked Potential
SSEP is a signal that is detectable on EEG and is generated in a time-locked fashion in response to a specific applied sensory input, typically a cutaneous electrical stimulation (i.e., of a peripheral sensory nerve, but also of a cranial nerve with a sensory pathway). As a result, an intact neural pathway from the periphery to the cerebral sensory cortex is essential for a signal to be generated. This monitoring modality has application in any surgical procedure that may jeopardize this pathway. Specifically, spine surgery in which the dorsal column of the spinal cord may be placed at risk is a particularly appropriate application, but it may also be used during other procedures such as craniotomy and carotid surgery where any part of the pathway may be subjected to ischemia or surgical retraction.
Because of the presence of spontaneous EEG activity, a single peripheral stimulus, which generates cortical activity of relatively low amplitude, would not be detectable amidst the background noise. Summation followed by signal averaging of repetitive stimuli is therefore necessary in order to extract meaningful signals.
Stimulation is typically done in the regions of the median nerve, ulnar nerve, and posterior tibial nerve to generate predictable and reliable signals. However, in theory, any sensory nerve could be used to generate SSEP. The SSEP is described by its polarity (the direction of the wave deflection) and its latency (the time required for a signal to be detected after the stimulus has been applied), and is quantified by both the amplitude of that signal and its latency. For example, N20 is the SSEP generated via stimulation of the median nerve that is expected to have a latency of approximately 20 ms and a negative displacement (Fig. 36-8).
FIGURE 36-8. Representative tracings of multiple modalities of sensory evoked potential. BAEP, brainstem auditory evoked potential; ms, millisecond; n, nerve; SSEP, somatosensory evoked potential; VEP, visual evoked potential.
Disruption of the neural pathway at any point will result in complete loss of SSEP. More commonly, ischemia, not mechanical disruption, is the intraoperative insult. As a result of ischemia, the amplitude of the signal decreases and the latency increases. A 50% decrease in signal amplitude is generally accepted as clinically significant, as is a 10% increase in latency.
Brainstem Auditory Evoked Potential
BAEP is a specialized type of sensory evoked potential. Instead of an electrical stimulus applied to a somatosensory nerve, a standardized sound (click) is applied to the eighth cranial nerve via the auditory apparatus. A recognized series of peaks are generated with this technique, where the latency of each peak has significance with respect to the integrity of various parts of the auditory pathway. Although this monitoring modality is specific to cranial nerve VIII, and is particularly useful in acoustic neuroma surgery, it may be used during any surgical procedure around the brainstem to infer its integrity, although such use is associated with both low sensitivity and specificity.
Visual Evoked Potential
VEP signals are generated via light stimulation of the retina. Typically, goggles that emit LED lights are worn. Although this modality is particularly appealing to monitor the integrity of the optic nerve in settings in which visual loss is a concern, such as in prone spine surgery, the signals are not robust. They are difficult to record in a consistent fashion during anesthesia, although it appears to be more stable during propofol compared to inhalation anesthesia. Research is ongoing with respect to its intraoperative use, particularly with regard to its interpretation.
Motor Evoked Potential
MEP monitoring is different from the other evoked potential modalities described thus far. Whereas SSEP, BAEP, and VEP provide information about ascending sensory neural pathways (i.e., from the periphery to the cerebral cortex), MEP evaluates descending motor pathways (i.e., from the cerebral cortex, past the neuromuscular junction, to peripheral muscle groups). This difference allows MEP to complement SSEP, particularly in the setting of spine surgery, in which the two modalities provide information about the integrity of anatomically different areas of the spinal cord. With MEP, the stimulus is applied in a transcranial fashion over the motor cortex. The deflection, essentially an electromyographic signal, is then detected by electrodes embedded in the muscle belly. Although theoretically the stimulus can be delivered with either a magnetic or electrical source, transcranial magnetic stimulation is obliterated under anesthesia. The transcranial electrical signal is usually delivered as a rapid train of four or more stimuli, the voltage of which is adjusted to achieve adequate signals in both the upper and lower extremities. The MEP is typically detected at the thenar eminence and the abductor hallucis muscle. Transcranial electrical MEP is of substantially greater magnitude compared with SSEP, and signal averaging with repetitive stimuli is therefore not required. However, it is very sensitive to anesthetic agents, particularly inhalation anesthetics. Its amplitude can be augmented by increasing the transcranial voltage, or the number of stimuli in the train. The stimulus can cause patient movement, so MEP signals are typically obtained intermittently at points during the surgery when slight patient movements are not problematic. A bite block is mandatory to prevent injury to the tongue during transcranial stimulation.
With MEP, latency of the signal is somewhat unreliable, and not typically used to make clinical decisions. Decision making is based on amplitude alone, where a 50% decrease is considered significant (Fig. 36-9). Although MEP can be used during any spine or intracranial surgical procedure, it is becoming increasingly used during cervical spine surgery.
FIGURE 36-9. Representative tracing of motor evoked potential (MEP) recorded from the thenar muscles in response to transcranial electrical stimulation.
MEP signals are much more sensitive to volatile anesthesia than SSEP. Although there is some evidence that MEP signals are adequate during desflurane anesthesia, more research on the efficacy of this technique is required, and total intravenous anesthesia is the preferred technique when MEP monitoring is required.25 Some centers use partial neuromuscular blockade, but most centers avoid muscle relaxants altogether with MEP in order to avoid compromise of the signal.26
Spontaneous Electromyography
Spontaneous electromyography (EMG) is different from other evoked potentials in that a signal is not intentionally generated through stimulation at some point in a known neural pathway. Instead, it is a continuous recording of EMG activity in the muscle of regions innervated by nerve roots around which surgeons are working. Its purpose is to detect injury to those nerve roots by the surgical procedure. Impingement on a nerve root by an instrument will cause immediate motor activity that is easily detectable, which may allow the surgeon to modify his or her technique. Although spontaneous EMG is a robust signal that is tolerant of various anesthetic techniques, muscle relaxant must be avoided. Spontaneous EMG is frequently used during cervical and lumbar spine surgery where the brachial plexus and lumbosacral plexus are encountered.
Cranial Nerve Monitoring
Surgery in the posterior cranial fossa and adjacent to the brainstem places the surgeon in close proximity to cranial nerves. Although cranial nerve VIII can be monitored with BAEP as discussed earlier, several other cranial nerves can be monitored as well. Generally, only the integrity of nerves with motor components can be detected, either through spontaneous EMG or through EMG evoked by local electrical stimulation. These include cranial nerves V, VII, IX, X, XI, and XII. Cranial nerve X is usually monitored via special monitoring endotracheal tubes embedded with electrodes near the cuff.
Influence of Anesthetic Technique
As mentioned previously, anesthetic agents can have a profound influence on the amplitude and latency of evoked potentials. For instance, the quality of signals obtained with SSEP monitoring depends on the anesthetic agents used. Signals are obtainable under volatile anesthesia, but the anesthetic is typically kept at sub-MAC (minimum alveolar concentration) doses to avoid degradation in quality (increase in latency and decrease in amplitude), as amplitude of SSEP signals are depressed by volatile agents in a dose-related manner; they are recordable during low dose and obliterated with high doses. Potent volatile anesthetics should not be combined with nitrous oxide, as this technique will further compromise quality. The signals are unaffected by opioids, and opioid infusions are frequently used to facilitate low-dose volatile anesthesia. Signal quality is also excellent under intravenous anesthesia with propofol. Ketamine has been shown to enhance evoked potential monitoring. Dexmedetomidine infusion has also been used as an adjunct, allowing a reduction in propofol dose, but its effects on MEP remain controversial, with some studies reporting a lack of effect while some reports suggest a deleterious effect.27,28
To summarize the influence of anesthetic agents on evoked potential monitoring, general statements can be made.
1. Inhalation agents including nitrous oxide generally have more depressant effects on EP monitoring than intravenous agents.
2. Cortical EP with long latency involving multiple synapses are exquisitely sensitive to the influence of anesthetic while short latency brainstem and spinal components are resistant to anesthetic influence. Thus, BAEP can be recorded under any anesthetic technique, whereas VEP and SSEP are very sensitive.
3. Monitoring of MEP and cranial nerve EMG in general preclude the use of muscle relaxants, although the use of a short-acting neuromuscular blocking agent for the purpose of tracheal intubation is not contraindicated as its effect usually wears off before monitoring and surgery begins.
4. MEP is exquisitely sensitive to the depressant effects of inhalation anesthetics including nitrous oxide. Although it can be recorded with low-dose agents, the signals are so severely attenuated that this practice is generally not advisable. Total intravenous anesthesia without nitrous oxide is the ideal anesthetic technique for monitoring of MEP. Ketamine may enhance the amplitude of MEP, while dexmedetomidine may have either negligible or some depressant effects.
5. Opioids and benzodiazepines have negligible effects on recording of EP.
6. Propofol and thiopental attenuate the amplitude of virtually all modalities of EP but do not obliterate them. SSEP and MEP can be monitored even during burst suppression induced by these agents. BAEP can be recorded with any anesthetic technique.
7. During crucial events in which part of the central neural pathway is specifically placed at risk by surgical manipulation, as in placement of a temporary clip during aneurysm surgery, change in “anesthetic depth” should be minimized to avoid misinterpretation of the changes in EP recorded.
8. Ketamine and etomidate have been reported to enhance the quality of signals in patients with weak baseline SSEP signals, although the clinical significance and interpretation of signals obtained under these circumstances remain unclear.
Cerebral Perfusion
Although adequate CBF does not guarantee the well being of the CNS, it is one factor that is essential to its integrity. Measuring CBF is therefore an attractive method of monitoring the CNS. Currently available techniques for quantitative measurement of CBF are not practical as an intraoperative monitor, but other methods for looking at relative changes in CBF do lend themselves to use in the operating room. Transcranial Doppler ultrasonography (TCD) and laser Doppler flowmetry are examples. Furthermore, as adequate CBF depends on an appropriate CPP, measuring ICP may be useful in certain patients to ensure conditions are adequate for sufficient CBF. Finally, numerous other modalities that evaluate CBF and that may not be practical in the operating room are used commonly in the perioperative setting.
Laser Doppler Flowmetry
Laser Doppler flowmetry is a technique that measures cortical blood flow in a small region of the brain adjacent to the placement of the device. Although it is useful for detecting relative changes in CBF, its utility is limited by several factors. First, it requires a burr hole for placement, which prevents its use in most patients. Second, it measures flow in only a small region of the brain; it could miss hypoperfusion in any area of the brain not directly monitored. Accuracy is also affected by movement and the presence of underlying major vessels. Because of these limitations, laser Doppler flowmetry has found limited applications.
Transcranial Doppler Ultrasonography
TCD is a noninvasive monitor for evaluating relative changes in flow through the large basal arteries of the brain (i.e., the circle of Willis). TCD does not measure flow directly, and therefore cannot provide information regarding absolute CBF. TCD measures flow velocity (Fig. 36-10), which is directly proportional to the flow if the diameters of these large vessels are constant. Except in well-known circumstances such as cerebral vasospasm following aneurysmal subarachnoid hemorrhage, these vessels are thought to be conductance vessels, where diameters of the basal arteries are stable.29 Pressure autoregulation and CO2 reactivity of CBF occurs via changes in arteriolar diameter distal to these large vessels.
FIGURE 36-10. Transcranial Doppler tracing with release of cross-clamp during carotid endarterectomy. The resultant hyperemia is accompanied with evidence of air emboli (vertical streaks on the tracing). MCA, middle cerebral artery; ICA, internal carotid artery.
Although the vessels that can be evaluated with TCD include the middle cerebral artery, internal carotid artery, anterior cerebral artery, posterior cerebral artery, ophthalmic artery, vertebral artery, and basilar artery, not all of these vessels can be monitored continuously during surgical procedures. Many of these vessels can only be evaluated with a hand-held TCD probe, which is useful for providing a brief snapshot of flow velocity in that vessel. A commercially available device for fixation of the TCD probe is essential for continuous monitoring. These devices are available either as a headband or as a rack that remains attached via fixation points on the bridge of the nose and in bilateral auditory canals. With these devices, flow velocity in the middle cerebral artery can be continuously evaluated.
In addition to the measurement of flow velocity, TCD is useful for detecting emboli. Microembolic signals can be generated by the passage of either gas or particulate matter (Fig. 36-11). The former is likely to occur as a result of venous air embolism (VAE), particularly if the patient has a patent foramen ovale, while the latter may occur during the manipulation of an atheroma in a neck vessel or as the result of thrombus formation and dislodgement on a vascular dissection.
FIGURE 36-11. Particulate emboli seen on transcranial Doppler in a patient with symptoms of transient ischemic attacks consistent with right carotid artery territory embolization. The emboli are denoted by the arrows. MCA, middle cerebral artery; PW, pulse wave.
Specific applications for intraoperative use of TCD include CEA, nonneurologic surgery in patients with TBI, and surgical procedures requiring cardiopulmonary bypass. There are also numerous indications for TCD in the perioperative setting.
Perhaps the most important use of TCD is in the neurocritical care unit, where it is used to monitor the development of vasospasm in patients who have suffered a subarachnoid hemorrhage. It has also been used for the measurement of cerebral autoregulation in patients with TBI, vasomotor reactivity in patients with occlusive vascular disease, noninvasive measurement of ICP, determination of intracranial circulatory arrest and confirmation of brain death.30–33
Intracranial Pressure Monitoring
Although monitoring ICP does not provide direct information about CBF, it allows the derivation of CPP, which must be in an appropriate range in order for CBF to be adequate. CPP is defined as the difference between MAP and ICP. In other words, it is the net pressure acting to move blood through the cerebral vasculature (assuming ICP is greater than right atrial pressure). CPP and CBF are not expected to be proportional, as there are other factors determining CBF (discussed elsewhere). In fact, within a physiologic range of CPP, CBF should remain approximately constant. However, a CPP that is too low will result in cerebral ischemia and CPP that is too high will cause hyperemia.
ICP monitoring is recommended in all salvageable patients with severe TBI (GCS ≤ 8) and an abnormal CT scan (hematomas, contusions, swelling, herniation, or compressed basal cistern), and in patients with severe TBI with a normal CT scan if two or more of the following features are noted at the admission: Age >40 years, unilateral or bilateral motor posturing, or SBP <90 mm Hg. However, patients who present to the operating room in the acute stage of injury will seldom have an ICP monitor in place, and the presence of elevated ICP must be inferred from the medical history, physical examination, and CT scan.
When ICP is high and CPP is low, interventions can target either ICP or MAP in order to restore a favorable balance of the two. Ideally, ICP should be maintained under 20 mm Hg. Interventions to lower ICP include suppression of cerebral metabolic activity, positional changes to decrease cerebral venous blood volume, drainage of CSF, removal of brain water with osmotic agents such as mannitol, and if absolutely essential, mild-to- moderate hyperventilation to further decrease CBV. MAP is raised via adequate intravascular resuscitation and with a vasopressor as needed. The goal CPP in TBI is >50 to 60 mm Hg.34
Other Modalities
Although seldom employed in the intraoperative setting, CT perfusion, single-photon emission computed tomography, positron emission tomography, and cerebral angiography all have roles, experimental or clinical, in the evaluation of CBF. However, these techniques are frequently used preoperatively and postoperatively in neurosurgical patients. On the other hand, intraoperative angiography is frequently used during neurovascular surgery to confirm placement of an aneurysm clip or to verify complete obliteration of an arteriovenous malformation (AVM). More recently, this has been superseded by indocyanine green videoangiography, as this obviates the need for radiation.35
Cerebral Oxygenation/Metabolism Monitors
A number of invasive and noninvasive monitors provide insight into the metabolic state of the brain and the level of tissue oxygenation of the brain, both of which reveal the balance between blood supply and metabolic demands.
Near-infrared Spectroscopy
Near-infrared spectroscopy (NIRS) is a noninvasive method of detecting the oxygenation of cerebral tissue. It is based on reflectance spectroscopy; it measures the light reflected from chromophobes in the brain (hemoglobin) to derive the regional oxygen saturation. In this manner, it provides an indication of the balance between flow and metabolism. Typically, a sensor is applied to the forehead (over hairless skin). A light signal is transmitted through the skin, skull, and meninges into the cerebral cortex. A complex analysis of the reflected light allows calculation of the oxygenation of blood in the cortex, which is a mix of venous and arterial blood. Falling saturation indicates a decline in cerebral perfusion. Exact thresholds for concern relate to the specific device used for this purpose.
Bilateral monitoring is particularly attractive for procedures that place a single hemisphere at risk for ischemia, such as CEA carotid surgery. In this setting, the development of significant asymmetry in cerebral oxygenation could be used as an indicator for the need for a shunt.
Individual variations in extracranial tissue (hence contamination), arterial to venous blood volume ratio, systemic blood pressure, PaCO2, hematocrit, and regional CBV are factors that can influence cerebral tissue oxygenation, and this creates potential difficulties when attempting to establish a consensus value for NIRS-derived “thresholds” for ischemia/hypoxia.36 It is generally accepted that normal range varies between 60% and 75%, with a coefficient of variation of almost 10%.36,37
The applicability of NIRS in brain injury monitoring is as yet to be defined and there are no data to support the widespread application of NIRS to monitor cerebral oxygenation routinely during anesthesia and surgery. Ironically NIRS has found most acceptance in cardiac anesthesia, where access to the forehead for placement of the sensor is less of a problem than in neuroanesthesia. Nevertheless, it is a promising technology that, as advances in design are made, may find increasing application in the field of neuroanesthesia.
Brain Tissue PO2
The brain tissue PO2 (PbtO2) monitor is an invasive probe that is inserted through a burr hole into the brain parenchyma, typically in conjunction with a fiberoptic ICP monitor. As a result, this monitor is used most commonly in patients with TBI. It measures oxygen tension in the surrounding brain. An adequate CPP in such a patient is encouraging, but it does not guarantee adequate blood supply to meet the metabolic needs of the brain. PbtO2 complements ICP information in that it provides insight into oxygen delivery. A low PbtO2 is indicative of inadequate oxygen delivery to that area of brain. A level of 15 mm Hg is concerning for cerebral hypoxia, and warrants intervention in TBI.38,39 In patients with TBI, PbtO2 has been shown to correlate well with the treatment effects and outcome.40
Its major limitation is that the information it provides reflects oxygenation at the local level—in proximity to the probe—meaning that an adequate PbtO2 at that location may not guarantee adequate oxygen delivery to other regions of the brain.
Interventions to raise the PbtO2 must either target oxygen delivery or oxygen consumption. In order to improve oxygen delivery, FIO2-inspired oxygen can be increased, but treating anemia makes more sense from a physiologic perspective. Decompressive craniectomy may improve perfusion. Decreasing oxygen requirements can be accomplished by metabolic suppression with propofol or a barbiturate, as well as by treating hyperthermia with external cooling, acetaminophen, and when appropriate, nonsteroidal anti-inflammatory medications.
Jugular Venous Oximetry
Although PbtO2 gives a local view of the balance of oxygen supply and demand, jugular venous oximetry provides that same information for a larger portion, if not the complete, brain. For this monitor, a catheter is inserted into the jugular vein in a retrograde fashion so that its tip sits at the base of the skull in the jugular bulb. This allows continuous pressure monitoring as well as intermittent withdrawal of a jugular venous blood sample for gas analysis. Continuous monitoring can be achieved using an oximetry catheter inserted via a conduit sheath. Confirmation of location can be made with a lateral cervical spine film.
For best representation of the metabolic state of the brain, the catheter should be placed in the dominant jugular vein, most commonly the right side. In patients who have had a cerebral angiogram, the venous phase of the study will provide information on dominant venous drainage. Often the intra-arterial contrast will drain almost exclusively through one jugular vein, regardless of the side of injection. Side dominance can also be predicted using ultrasound where the dominant vein may be larger. In the absence of this information, the right side is preferred.
Pressure transduction of the jugular bulb catheter allows comparison with the central venous pressure to rule out potential venous obstruction. In a supine patient with a neutral neck position, there should be no pressure gradient between the tip of the jugular bulb and the central venous catheter. Although rare, a significant gradient (>4 mm Hg) can occasionally develop during positioning if there is significant twisting or bending of the neck. This gradient indicates venous obstruction, potentially causing brain edema, or ischemia. The head should be repositioned until the gradient resolves.
Blood gas analysis of the sample provides several useful parameters. The saturation of jugular venous blood (SjvO2) demonstrates whether CBF is sufficient to meet the cerebral metabolic rate for oxygen (CMRO2) of the brain. A normal value is in the 65% to 75% range. In TBI, SjvO2 below 50% for more than 10 minutes is undesirable and associated with poor outcome.41 However, it has low sensitivity, and a study using PET scan indicates that a relatively large volume of tissue must be affected, approximately 13%, before SjvO2 levels decreased below 50%.42 Intraoperative hyperventilation will lower SjvO2 as it decreases CBF. In the setting of a nontraumatized brain that is exposed to moderate hyperventilation for the duration of a neurosurgical procedure, the acceptable level for SjvO2 is unknown. In the absence of other demands, it is reasonable to guide intraoperative hyperventilation by maintaining SjvO2 >50%. It is essential that blood samples from the retrograde catheter be drawn slowly to avoid contamination from noncerebral venous blood.43
Measurement of simultaneous arterial and jugular venous samples allows the determination of lactate output from the brain, the presence of which indicates occurrence of anaerobic metabolism. The obvious disadvantage to jugular venous oximetry is precisely the opposite of the shortcoming for PbtO2, in that it is a global monitor that could easily miss small areas of regional ischemia. The two monitors may be complementary in the setting of TBI. Intraoperatively, jugular venous oximetry is used routinely in some centers that specialize in neurosurgical procedures.
CEREBRAL PROTECTION
Cerebral ischemia and/or hypoxia leads to neuronal death in multiple settings. For example, ischemic stroke, TBI, and cerebral vasospasm following subarachnoid hemorrhage. Efforts to avert neurologic insult, using medications or through the manipulation of physiologic parameters, have met with meager results. In the setting of ischemic stroke, for example, thrombolysis may restore perfusion and decrease infarct size, but it may also lead to expansion of the infarct, edema, and even hemorrhage as a result of ischemia-reperfusion injury. In general, a protective strategy that is effective in experimental cerebral ischemia has not been found to be useful in the clinical setting. Of recent advances that are intriguing and controversial, none matches that generated by the concept of cerebral protection by mild or moderate hypothermia.
Persons suffering out-of-hospital cardiac arrest have been shown to have improved neurologic outcome if they are made mildly hypothermic following resuscitation.44,45 Therefore, it would seem that mild hypothermia is protective against global ischemia/hypoxia at least in the setting of cardiac arrest.
One problem with most settings in which cerebral ischemia is encountered is that the therapeutic intervention can be applied only after the insult has occurred, that is, during the reperfusion phase. Little opportunity exists to intervene before the ischemic event. However, the operating room is a unique environment in this respect. Many ischemic insults that patients suffer in the operating room are iatrogenic and anticipated. A temporary aneurysm clip on the middle cerebral artery is an example of a focal ischemic insult that could be predicted, and a brief period of circulatory arrest induced with adenosine to facilitate clipping of a basilar artery aneurysm is an example of a global insult. The value of anticipating such events is that it allows the anesthesiologist to intervene in advance.
Despite the luxury of planning the intervention for the ischemic insult, the options anesthesiologists have for cerebral protection are few and the evidence for benefit is modest; much of this evidence has been extrapolated from animal research. Each technique will be examined in detail here.
Ischemia and Reperfusion
Ischemic insult to the brain results in energy failure. The brain depends on a continuous supply of glucose and O2 to support aerobic metabolism, generation of adenosine triphosphate (ATP), and maintenance of cellular function. When this nutrient supply is interrupted, ATP is depleted. Cellular processes, such as those that maintain cellular membrane integrity, fail. It is reasonable then to attempt to minimize ischemic insult by lowering cerebral metabolic rate, thus decreasing the likelihood of exhausting ATP reserves during the period of ischemia. This has been the traditional paradigm for approaching the subject of intraoperative neuroprotection.
Unfortunately, further damage occurs as a result of processes that are initiated during the reperfusion stage. The reperfusion injury may be mediated via the generation of toxic oxygen species, release of excitotoxic amino acids such as glutamate, up-regulation of nitric oxide synthase, and initiation of cellular apoptosis. Further therapeutic interventions would need to target these pathways as well to provide protection. A shift in the focus of neuroprotection from metabolic suppression to targeting ischemic cascades has recently been advocated.46
Hypothermia
It is important to distinguish mild/moderate and profound hypothermia, as they have very different practical considerations and they likely modify cerebral function in different ways.
Profound hypothermia is well known for its neuroprotective effects. Anecdotes of successful resuscitation of hypothermic drowning and avalanche victims with good neurologic recovery have been reported.47,48 Furthermore, extensive use of deep hypothermia with circulatory arrest has been used intraoperatively for the repair of aneurysms of the thoracic aorta and for cerebral aneurysms.49,50 When core body temperature is <20°C, and the brain is <15°C, circulatory arrest of <30 minutes appears to be well tolerated. This level of hypothermia not only decreases cerebral activity, but it also decreases the energy required for cellular housekeeping. The practical constraints against using deep hypothermia in settings in which cerebral ischemia is anticipated are numerous. Foremost is the need for cardiopulmonary bypass during the cooling and warming portion of the procedure. Hypothermia-induced coagulopathy is another concern during surgical procedures in the cold patient. Despite the drawbacks to this technique, it remains a reasonable anesthetic option to provide protection for the brain and other organs when the surgical procedure necessitates circulatory arrest.
Mild hypothermia (33° to 35°C) not only decreases cerebral metabolism, but likely modulates the immune and inflammatory response to ischemia, thus affecting the reperfusion portion of the injury as well. Animal studies have shown improved neurologic function following resuscitation from arrest.51 This promising result in animals was later confirmed by two independent studies in humans, demonstrating that induction of hypothermia in cardiac arrest patients improved the outcome.44,45
Although mild hypothermia is clearly beneficial in the setting of cardiac arrest, cerebral ischemia due to an arrest is an uncommon occurrence in patients under anesthesia. In contrast, the cerebral ischemia frequently encountered by the anesthesiologist is focal in nature because of the temporary occlusion of a cerebral vessel. Although there is considerable evidence in rats that mild hypothermia is beneficial here too, there is a paucity of evidence in humans. In fact, a large multicenter study (IHAST II—Intraoperative Hypothermia for Aneurysm Surgery Trial) evaluating patients undergoing cerebral aneurysm surgery found no benefit with mild intraoperative hypothermia.52 However, the study was not designed to study patients at the highest risk—that is, those who had undergone temporary occlusion for more than 20 minutes. Although the sample size is small, a post-hoc analysis of these at-risk patients suggest that there is a trend for either hypothermia or metabolic suppression to improve outcome.53
Nevertheless, hypothermia remains our most promising intervention for cerebral protection. There is a compelling physiologic rationale for its use, a clearly demonstrated effect in animals, and human data showing benefit in the setting of cardiac arrest. Unfortunately, inadequate evidence exists in humans outside cardiac arrest to recommend its routine use in the neurosurgical patient.
Despite the lack of evidence to support hypothermia in humans for cerebral protection, there is ample evidence that hyperthermia is associated with worse outcome in the setting of ischemic stroke, subarachnoid hemorrhage, cardiac arrest, and TBI.54–57 A common extrapolation from these studies is the belief that concomitant hyperthermia and cerebral ischemia is deleterious. However, it is important to consider that these studies demonstrate an association, not a causation, of poor outcome from fever. Nevertheless, it would seem reasonable to avoid hyperthermia and treat fever aggressively in any setting in which the brain is at risk.58
In the operating room, during neurosurgical procedures in which the brain is at risk for ischemic insult, a goal temperature of 35° to 36°C is reasonable. Mild hypothermia (33° to 35°C) may be appropriate in many patients with a planned period of temporary focal ischemia (as in temporary occlusion for aneurysm clipping) even recognizing that there is currently a lack of solid evidence to support this therapy. Finally, deep hypothermia (<20°C) is appropriate in any situation in which a prolonged cardiac arrest is required.
Medical Therapy for Cerebral Protection
Volatile and intravenous anesthetic agents decrease cerebral metabolism, and thus seem like appropriate candidates for cerebral protection. However, evidence that the level of metabolic suppression does not correlate with the degree of protection has eroded the traditional belief in the mechanism of protection.59 Nevertheless, numerous animal studies have found protective effects of volatile anesthetics, particularly isoflurane, in mitigating mild-to-moderate ischemic insult, although this effect may only be short-lived.60–63 This effect may exist when applied during the insult, but also may be effective when administered prior to the insult as a preconditioning therapy.64,65
Barbiturates, such as thiopental, have been extensively researched in regard to cerebral protection. They have been shown to have at least short-term benefit on focal cerebral ischemia, while benefit in global ischemia remains controversial.66–74 This effect may be mediated through a reduction in glutamate activity and intracellular calcium, an increase in γ-aminobutyric acid (GABA) activity, as well as N-methyl-D-aspartate (NMDA) antagonism.75,76 Propofol likely has similar protective effects through its action on GABA receptors, as well as via free radical scavenging and limiting lipid peroxidation.77,78 Again, the durability of this protection is unknown.
Current opinion is that anesthetic neuroprotection is primarily mediated through prevention of excitotoxic injury, not through termination of apoptotic pathways; it thus delays neuronal death and leaves a greater temporal window for intervention.61 Without other therapeutic options to prevent eventual cell death, outcome is unlikely to be improved, save for perhaps the setting of mild ischemic insult in which apoptotic pathways are not initiated. Sufficient evidence in humans to guide clinical interventions, apart from modest hypothermia in cardiac arrest, is difficult to obtain.45,79 Clinically, barbiturates and propofol are used intraoperatively to achieve metabolic depression as evidenced by a burst suppression EEG pattern, and although not statistically significant, results from IHAST II suggest that patients undergoing temporary occlusion for >20 minutes may benefit from metabolic suppression therapy.53
Glucose and Cerebral Ischemia
Although hyperglycemia has long been recognized as a frequent occurrence in critically ill patients, it was commonly viewed as benign or even beneficial.80 Hyperglycemia could facilitate cellular uptake of glucose through noninsulin-dependent mechanisms, and thus may benefit cellular metabolism. A subsequent recognition of its association with worse outcome in many settings, including acute coronary syndrome, stroke, TBI, and critical illness, forced the medical community to reconsider the burden of hyperglycemia.81–86 Furthermore, animal studies suggested that hyperglycemia in the setting of both cerebral and myocardial ischemia increased infarct size.87,88
Although considerable evidence accumulated suggesting harm associated with hyperglycemia, evidence for benefit with normalization of serum glucose using insulin has been somewhat controversial. The most influential literature is from the intensive care unit (ICU) setting, not the operating room. A prospective study in surgical ICU patients (predominantly after cardiac surgery) showed that mortality and morbidity benefit with tight glycemic control (80 to 110 mg/dL).89 This study spurred an unfettered enthusiasm for aggressive treatment of hyperglycemia, changing practice not only in the surgical ICU but the medical ICU, and in many cases, the operating room. However, a subsequent study evaluating this therapy in a much sicker medical ICU population showed no overall mortality benefit.90 In fact, subgroup analysis revealed increased mortality in patients who stayed in the ICU <3 days, with an improvement only in those who had a longer ICU stay. In the heterogeneous patient population who present for neurologic surgery, with operative times of several hours, not several days, it is inappropriate to extrapolate conclusions from a body of controversial ICU literature to the anesthetic environment, particularly when there is evidence for harm with short durations of therapy. Furthermore, a prospective study of intraoperative insulin therapy in cardiac surgery patients further eroded the basis for translating this ICU literature to the operating room; the insulin group had a higher incidence of death and stroke.91
Despite our reluctance to embrace intraoperative tight glycemic control given the current literature, it is worthwhile to consider the patient undergoing cerebrovascular surgery in particular. Given the preponderance of evidence that hyperglycemia and cerebral ischemia in combination are harmful, changing practice in these patients may be warranted. Hyperglycemia on the day of surgery for CEA is associated with worse outcome.92 And, patients who suffer from an ischemic stroke have an improved outcome if their glucose is treated aggressively.93 Therefore, it may be appropriate to treat neurosurgical patients who will have a period of cerebral ischemia due to temporary vascular occlusion differently from other neurosurgical patients. Better glycemic control is a reasonable goal in these patients, aiming for a range of 140 to 180 mg/dL however, we cannot state at this time that this intervention is neuroprotective.
Promising Areas of Research
Continued research in the various excitotoxic and apoptotic pathways that lead to cell death with cerebral ischemia is essential to bring promising interventions to the clinical arena. It is likely that only a multimodality approach will create durable meaningful cerebral protection.61 Mild to moderate hypothermia continues to hold promise, given its efficacy in experimental focal ischemia. Several additional medical interventions show potential as well. Statins, which inhibit 3-hydroxy-3-methylglutaryl-coenzyme A (HMG-CoA) reductase, have nonlipid lowering effects such as improved endothelial function, as well as antithrombotic and anti-inflammatory activity, which may be neuroprotective.94 Furthermore, the nonhematopoietic effects of erythropoietin include mitigation of lipid peroxidation and prevention of apoptosis.95 Whether these medications will offer any benefit to neurosurgical patients remains to be determined.
A Practical Approach
In the absence of compelling evidence in humans regarding the benefit of one practice over another, it is difficult to present firm guidelines with respect to the prevention of intraoperative ischemic insult. Maintaining optimal systemic and cerebral hemodynamics, as well as oxygenation, remain the most important principles. Beyond these, for patients undergoing surgical procedures with an anticipated period of temporary focal cerebral ischemia such as cerebral aneurysm surgery or cerebrovascular bypass procedures, either volatile anesthesia or an intravenous technique can be used. It is reasonable to administer additional propofol or thiopental prior to vessel occlusion. Optimally this intervention should be guided by EEG monitoring with the goal of achieving burst suppression nearing a 50% ratio. A total intravenous anesthetic technique is preferred when maximal brain “relaxation” is desired. Euglycemia, or near euglycemia prior to vessel occlusion is desirable (140 to 180 mg/dL), but frequent glucose checks are essential throughout the anesthetic to avoid episodes of hypoglycemia if insulin is administered. Finally, hyperthermia should be avoided during this time, with the temperature kept at or below 36°C.
ANESTHETIC MANAGEMENT
Preoperative Evaluation
Risk stratification is indicated for neurologic or spine surgery to assess the patient’s neurologic condition, as well as the inherent risk of the surgical procedure. For a smooth transition from patient referral to surgical intervention, dynamic communication between neurosurgeons, anesthesiologists, the preanesthesia clinic, neurophysiologists, and the laboratory is essential.96,97
Preoperative assessment allows identification of modifiable risk factors, optimization of the patient’s condition, explanation of the risks and formulating the best possible anesthetic plan to improve patient safety, optimize resource utilization and increased patient satisfaction. A thorough history may be difficult to obtain from patients whose disease has resulted in a neurologic decline, such as those obtunded from TBI. Prior medical records, discussion with family physicians and family members are both helpful in this context.
Perioperative cardiac outcome is influenced by urgency, magnitude, type, duration of surgery, associate blood loss, fluid shifts, and change in body temperature. The 2007 American College of Cardiology/American Heart Association guidelines has a simplified algorithm for considering whether a patient needs preoperative cardiac testing, such as stress echocardiography, or a nuclear medicine evaluation of myocardial perfusion.98 Spine and neurosurgical surgery fall into the intermediate-risk procedure category. The decision to perform a noninvasive cardiac test in patients with risk factors for coronary disease and poor functional status hinges on whether findings from that evaluation will affect management of the patient in the time before surgery. For neurologic patients with significant cardiac disease, current guidelines include delaying surgery for at least 2 weeks following simple balloon angioplasty, 1 month for a bare metal stent, and a full year for a drug-eluting stent. However, it is generally not feasible to delay most of the spine and neurosurgical procedures. For these patients joint consultation with cardiologists is essential. As for beta-blockers, the results of the POISE trial indicated that perioperative beta-blockade is recommended primarily in two types of patients undergoing intermediate-risk surgical procedures: Those already receiving a beta-blocker, and those who are at high risk for perioperative myocardial infarction due to documented reversible ischemia from a noninvasive study.99,100 Furthermore, patients previously receiving a statin should continue their statin in the perioperative period.
Further considerations in the preoperative visit should include issues that will affect choice of medications and anesthetic agents. Many patients presenting for spine surgery have weakness or paralysis that may present a contraindication to the use of succinylcholine. In addition, some neurosurgical patients may have suffered from a stroke resulting in a similar contraindication. Finally, many neurosurgical patients have been exposed to antiepileptic medications, which are known to induce liver enzymes and alter drug metabolism. Previous allergies or reactions to these medications, especially phenytoin, should be elucidated.
Induction and Airway Management
With the exception of some minimally invasive spine surgery procedures and awake craniotomies, placement of an endotracheal tube is essential for most surgical procedures of the brain and spinal cord.
During induction of anesthesia, hypotension, hypertension, and prolonged apnea should be avoided. A brief period of mild hypotension is frequently encountered following induction of anesthesia. Although most patients tolerate this transient phenomenon well, it should be aggressively avoided and/or treated in patients with brain injury in which any episode of hypotension may be associated with unfavorable outcome.101 Hypertension due to laryngoscopy, in contrast, is poorly tolerated by patients following aneurysmal subarachnoid hemorrhage, as systolic hypertension is thought to be a cause of recurrent hemorrhage from the aneurysm.102 Finally, apnea results in a predictable increase in PaCO2, and corresponding cerebral vasodilation. Although most patients tolerate the increase in CBV, patients with poor intracranial compliance may develop severe intracranial hypertension and decompensate from apnea, as well as suffer a decrease in cerebral perfusion.
TBI patients in particular are frequently intolerant of apnea. Unfortunately, many of these patients require a rapid-sequence induction. To further complicate matters, the presence of a cervical collar for known or suspected cervical spine injury may make intubation more difficult. Careful preparation for a difficult airway is essential. Furthermore, these patients may have concomitant injuries with significant blood loss that may predispose to systemic hypotension. Vigorous resuscitation with isotonic fluid and/or blood should be administered prior to induction and continued until the patient is euvolemic. A conservative dose of thiopental, propofol, or etomidate is appropriate for induction, with succinylcholine a reasonable choice as the muscle relaxant in the setting of acute injury.
Because patients with subarachnoid hemorrhage are at risk of rebleeding from hypertension, it is reasonable to place an arterial catheter for hemodynamic monitoring prior to induction. Unacceptable increases in blood pressure during laryngoscopy should result in discontinuing the attempt, returning to mask ventilation, and deepening the anesthesia. The latter can be accomplished either with a higher concentration of inspired volatile anesthetic, or a bolus of an intravenous agent such as propofol or remifentanil. In addition, esmolol (0.5 mg/kg) can be given prior to laryngoscopy to blunt the hypertensive response.
Most intravenous anesthetic agents (propofol, thiopental, and etomidate) are indirect cerebral vasoconstrictors, reducing cerebral metabolism (CMR) leading to a corresponding reduction of CBF while preserving autoregulation and CO2 reactivity. In contrast, ketamine has sympathomimetic properties. Its cerebral effects include an increase in CBF and ICP, but these are usually attenuated by the actions of other concurrently administered drugs.103–105 Etomidate decreases the cerebral metabolic rate, CBF, and ICP. At the same time, because of minimal cardiovascular effects, CPP is well maintained. However, it has been reported to reduce brain tissue oxygen tension, but the mechanism is unclear. Although changes in the EEG resemble those associated with barbiturates, etomidate enhances SSEPs and causes less reduction of MEP amplitudes than thiopental or propofol.106,107 The major limitation of etomidate is its known adrenal suppression effects. Even after a single induction dose etomidate has been shown to prolong hospital and ICU length of stay.108–110
The choice of muscle relaxant for use during induction deserves some consideration. Succinylcholine is contraindicated in patients with muscle denervation from stroke, myelopathy, or spinal cord injury (SCI) which results in up-regulation of acetylcholine receptor isoforms on myocyte membranes.111 In these patients, profound hyperkalemia may result from the use of succinylcholine which has the potential to lead to a cardiac arrest.112 However, it is generally safe to use succinylcholine in the first 24 to 72 hours after an injury, as well as, although controversial, after the spasticity is well established in about 9 months. In general a nondepolarizing muscle relaxant is appropriate for most neurosurgical patients to achieve acceptable conditions for tracheal intubation. Duration of action should be considered if MEP, spontaneous EMG, or cranial nerve monitoring is planned when adequate reversal is not possible. When used in appropriate doses, rocuronium (1.2 mg/kg) is comparable to succinylcholine (1 mg/kg) for rapid sequence induction.113 When available, profound neuromuscular blockade from rocuronium can be reversed with sugammadex, which currently awaits approval in the United States.114,115
Maintenance of Anesthesia
The primary considerations for maintenance of anesthesia include the type of neuromonitoring planned for the procedure, optimal brain relaxation, and the balance between adequate analgesia and the ability to assess neurologic function at the end of the surgical procedure.
Most neurosurgical and spine procedures can be performed using a balanced anesthetic with volatile agents and judicious doses of opioids to reduce volatile anesthetic requirements. For both SSEP monitoring and brain relaxation, less than one MAC of volatile anesthetic is desired. When selecting an opioid, an infusion of remifentanil, fentanyl, sufentanil, or alfentanil are all reasonable options. Because of its short context half-life, remifentanil is most appropriate for neurosurgical procedures in which tracheal extubation is planned at the end of the surgery and minimal residual effect is desired to facilitate neurologic examination.116 Other opioids with a longer duration of action are appropriate for spine surgery in which more durable analgesia following the procedure may be required. However, in most instances administration must be tapered toward the end of the procedure to avoid excessive analgesia and sedation that may interfere with prompt neurologic assessment.
Replacement of the volatile anesthetic with a continuous infusion of propofol is desirable in two settings. First, MEP monitoring virtually requires it to obtain optimal signal quality. Second, when brain relaxation is inadequate with a volatile anesthetic, propofol will provide better relaxation by further decreasing CBV.
The use of intraoperative muscle relaxant is controversial for neurosurgical procedures. It should be avoided during MEP, spontaneous EMG, or cranial nerve monitoring (except the VIIIth). However, it may be used during isolated SSEP monitoring. Some anesthesiologists are more comfortable managing a patient whose head is held in rigid fixation with muscle relaxant, but adequate anesthesia and avoidance of stimulating airway manipulations are far more important and should prevent unintended patient movement.
Dexmedetomidine, a highly selective α2-adrenoreceptor agonist, provides sedation without causing respiratory depression, does not interfere with electrophysiologic mapping and provides hemodynamic stability. It has been found to be particularly useful for implantation of deep brain stimulators in patients with Parkinson’s disease and for awake craniotomies, when sophisticated neurologic testing is required.117,118
Ventilation Management
Hypocapnic cerebral vasoconstriction provides the anesthesiologist with a powerful tool for manipulating CBF and CBV. Hyperventilation is routinely employed to provide brain relaxation and optimize surgical conditions. Because hyperventilation decreases CBF, it has the theoretical potential for causing or exacerbating cerebral ischemia. Clinically it has been associated with harm only in the early period of TBI, but it is still recommended to be avoided in all patients with TBI except when necessary for a brief period to manage acute increases in ICP.119,120 In the nontrauma population, it is not clear whether there is harm in mild-to- moderate hyperventilation, particularly for the duration of a typical anesthetic. As it appears to be well tolerated, it is reasonable during neurosurgical procedures to maintain a PaCO2 between 30 and 35 mm Hg. Further brain relaxation should be accomplished with other modalities, such as mannitol, hypertonic saline (HTS), or intravenous anesthesia. Should hyperventilation to a PaCO2 below 30 mm Hg be required, it might be appropriate to guide this therapy with jugular venous oximetry when available.
The duration of effectiveness of hyperventilation is also controversial as normalization of CBF, and consequently CBV, has been reported to occur within minutes. Clinically, the effects of CBV appear to be sustained during most neurosurgical procedures of modest duration.
Fluid and Electrolytes
To maintain adequate cerebral perfusion, adequate intravascular volume should be maintained. With perhaps the exception of healthy patients with an AVM, the aim should always be euvolemia or slight hypervolemia. For the care of the neurosurgical patient, a large volume of hypotonic fluids including lactated Ringer’s solution should not be used. Colloid has no proven advantage over crystalloid. Moreover, hetastarch can result in coagulopathy, and although low doses in healthy individuals is well tolerated, this may not be the case with patients undergoing intracranial procedures as the brain is rich in thromboplastin, the release of which may initiate coagulation abnormalities.
Both mannitol and HTS are effective in the treatment of intracranial hypertension.121–124 Hypertonic saline (3%) administration results in less dehydration and electrolyte disturbance, and may have anti-inflammatory actions with reduction in leukocyte adhesion and endothelial cell edema.125–127 With high-dose mannitol (2 g/kg), hyperkalemia can occur and serum potassium should be monitored.128 Because of the presence of the blood–brain barrier, movement of water into the intracellular and interstitial compartments of the brain from the vascular compartment is primarily dependent on the osmotic pressure, and not on the oncotic pressure. Consequently, to minimize brain edema, it is important to maintain serum tonicity. The most important osmotic species in blood is sodium, so it is prudent to check the serum sodium level on a regular basis in prolonged surgical procedures during which mannitol has been given. In addition to osmotic dehydration of the brain interstitium, other proposed benefits of hypertonic solutions include a reduction in blood viscosity, increasing erythrocyte deformability, and improved cardiac output and microcirculatory flow.129–135
Transfusion Therapy
There has been an increasing effort to conserve the limited resource of banked blood. While anemia is associated with increased in-hospital mortality, lower hospital discharge GCS score and discharge Glasgow outcome score, paradoxically RBC transfusion to maintain a higher hemoglobin (10 gm/dL vs. 7 gm/dL) is associated with more acute lung injury, longer ICU and hospital stay, and increased mortality.136 The lower limit of acceptable hemoglobin or hematocrit has not been well defined. The TRICC trial demonstrated that liberal transfusion targets in intensive care patients had higher mortality rates.136 Even though this study has been criticized for various reasons, including the poor representation of neurosurgical patients, it remains the best evidence to support avoidance of transfusion down to a hematocrit of approximately 21% except in the context of ongoing hemorrhage and possibly the early phase of resuscitation for septic patients.137 Despite the lack of evidence to support the practice, many who care for neurosurgical patients have advocated for more liberal transfusion practices to optimize oxygen delivery to the CNS.138,139 However, there is evidence to support a similar conservative transfusion threshold in both TBI and spine patients.140,141
Unfortunately, most of the evidence available on transfusion thresholds relates to critically ill but euvolemic patients. In the operating room with patients undergoing neurosurgical and spine procedures, ongoing hemorrhage may necessitate transfusion well before the hemoglobin falls to 7 g/dL (or a hematocrit of 21%).
A practical approach is to consider the rate of surgical blood loss. If it is slow, then it may be appropriate simply to maintain a normal intravascular volume with isotonic crystalloid solution or with an appropriate colloid, recognizing that albumin should be avoided in patients with TBI.142 Autologous predonation of blood, erythropoietic support, acute normovolemic hemodilution, intraoperative cell salvage, induced hypotension and the use of pharmacologic agents such as tranexamic acid, in addition to meticulous surgical hemostasis, have all been utilized with modest reductions in allogeneic blood transfusion rates during intracranial and spine surgery.143–145 However, such practice has not been widespread, as the benefits are marginal.
Monitoring of regional and/or global cerebral oxygenation may help determine the transfusion needs, and brain tissue oxygen tension as well as NIRS have been utilized for this purpose. Currently available evidence supports a hemoglobin threshold level of 8 to 9 g/dL. In the context of ongoing bleeding, lower levels (e.g., 7 g/dL) may result in brief periods of profound anemia, which may be linked to inadequate brain perfusion.146
Coagulopathy of trauma in patients with severe TBI has been noted to resolve more quickly than coagulopathy in patients without severe TBI. In trauma, patients with TBI when transfused with a high PLT:RBC ratio (platelet to red blood cell) experienced improved 30-day survival.147 A proposed mechanism may involve improved blood–brain barrier healing with activation of oligodendrocytes and repair of demyelination.148
Glucose Management
As discussed earlier, the combination of hyperglycemia and cerebral ischemia appears to be particularly deleterious.149–151 Although there is a paucity of evidence addressing the topic of intraoperative glucose management, logic would dictate that glucose should be normalized prior to periods of iatrogenic ischemia.
Tight glycemic control (80 to 110 mg/dL) with intensive insulin therapy has been shown to improve morbidity and mortality in critically ill patients.152,153 More recently, however, the findings of the NICE-SUGAR (Normoglycemia in Intensive Care Evaluation-Survival Using Glucose Algorithm Regulation) have suggested the use of intensive insulin therapy is associated with higher 90-day mortality.154 With regard to the neurosurgical population, intensive insulin treatment may result in increased variability in blood glucose leading to cerebral osmotic shifts and higher incidences of hypoglycemia, leading to worse outcome.155–160 Patients with neurologic injury may have poor correlation of systemic glucose with brain tissue glucose concentration. In this specific group, intensive insulin treatment may result in significantly lower brain tissue glucose concentration and the precipitation of brain energy crises. This risk is potentially greater in an anesthetized patient than an ICU patient, as the signs of hypoglycemia are masked by the anesthetic. Therefore, intraoperative glucose management with insulin requires frequent assessment of the serum glucose level. Patients who present for cerebrovascular surgery should have a preoperative glucose check. Those who are hyperglycemic should be started on an insulin infusion. Continuous closed-loop glucose control systems composed of pumps for the infusion of appropriate amounts of insulin and a glucose sensor for the detection and/or monitoring of glucose levels regulated by computerized algorithms, is an emerging method that could assist in the management of perioperative and inpatient hyperglycemia.
Intraoperative hyperglycemia is found to be common in adults undergoing urgent/emergent craniotomy for TBI and was predicted by severe TBI, the presence of subdural hematoma, preoperative hyperglycemia, and age ≥65 years.161 A target of 140 to 180 mg/dL is adequate in most patients to achieve this goal.
Emergence
The decisions that need to be made with respect to emergence from anesthesia of neurosurgical and spine surgery patients hinge on whether the patient is an appropriate candidate for tracheal extubation. To determine this, one must evaluate what has changed over the duration of the procedure with respect to the patient’s airway, oxygenation, ventilation and metabolic status. In addition, one must consider whether this patient will tolerate the hemodynamic changes that occur with extubation. Finally, postoperative plans, such as cerebral angiography, should be taken into account.
For extensive prone spine surgeries, significant dependent edema frequently occurs. Although the predictive value of a cuff leak from the endotracheal tube is poor in general, the combination of pronounced facial edema and an absent cuff leak following prone surgery should make one suspicious for upper airway edema.162–164 Delaying extubation of the trachea under these circumstances is prudent. In addition, intraoperative administration of intravenous steroids have not been found to be useful in prevention of airway edema and delayed extubation.165 Other factors that may delay extubation in these patients include the development of pulmonary edema and hypoxemia from fluid administration, as well as persistent hemodynamic instability.
For neurosurgical cases, usually the desire is to allow the patient to emerge from anesthesia and extubate the trachea as soon as possible after completion of the procedure. This pathway provides for an immediate neurologic examination and may obviate the need for postoperative CT scans. To facilitate emergence and extubation in the operating room, minimal use of opioids other than remifentanil is appropriate. Whether to give a longer-acting opioid, such as fentanyl or morphine, prior to emergence to treat postoperative pain is controversial. Opioids administration may delay emergence in a patient population that usually requires a relatively small amount of postoperative opioid for pain control.166 The antitussive properties of opioids may be desirable during emergence.
Avoidance of coughing and the hemodynamic changes associated with emergence is important for all neurosurgical patients, and particularly those at high risk for postoperative hemorrhage, such as patients who have just had resection of an AVM. Coughing due to irritation of trachea can be minimized with intravenous lidocaine (1 to 1.5 mg/kg). Instillation of 4% lidocaine in the cuff of the endotracheal tube for the duration of the procedure may achieve equivalent results.167 Labetalol, hydralazine, and nicardipine are all reasonable options for controlling hypertension on emergence.
COMMON SURGICAL PROCEDURES
Surgery for Tumors
The fundamental anesthetic considerations in tumor surgery are proper positioning of the patient to facilitate the surgical approach, providing adequate relaxation of the brain to optimize surgical conditions, and avoiding well-known devastating complications, such as VAE
. In addition, patients with large tumors resulting in significant intracranial hypertension are at risk of cerebral ischemia as well as herniation. Preoperative review of the level of consciousness and the CT scan should always be performed and the results taken into consideration in the anesthetic plan.
Patient positioning can be very challenging for any neurosurgical procedure, particularly for surgery in the posterior fossa. Lateral, park-bench, prone, and sitting positions are all used for surgical procedures in this region. When placing a patient in a complicated position for surgery, it is essential for the safety of the patient that all catheters and the endotracheal tube are secured particularly well. Ample help should be available at the time of positioning, particularly for obese patients. Padding adequately to avoid pressure necrosis is also essential. The head is typically secured in a Mayfield apparatus . Nothing should impinge on the nose, eyes, or chin.
As the sitting position confers the greatest risk for VAE, plans should be made for treating it, should it occur. The overall incidence of VAE is about 39% in posterior fossa surgery and 11% in cervical spine procedures. A multiorifice catheter can be placed in the right atrium to evacuate air. Its location can be confirmed either with the ECG or with echocardiography. A patent foramen ovale increases the risk of paradoxical embolism. However, transpulmonary passage of air has been described and its risk may be higher with volatile than intravenous anesthesia.168–170 Patients to be placed in the sitting position should be evaluated for a patent foramen ovale, either preoperatively or intraoperatively, and an alternate position should be considered for those who have one.
The structures in the posterior fossa, most notably the brainstem and cranial nerves, are particularly vulnerable and intolerant of surgical invasion. BAEP and cranial nerve monitoring are appropriate when the surgical procedure places the cranial nerves or brainstem at risk. SSEP and MEP monitoring can be used for any tumor resection, whether supra or infratentorial; these modalities may be particularly useful in surgeries that place specific tracts at risk.171,172
The brainstem is intimately involved in systemic hemodynamics, and surgery in that region may affect rapid changes in blood pressure and heart rate. Hemodynamic lability should be anticipated and treated during surgery in this region. Bradycardia can be treated with atropine, but it should also prompt communication with the surgeon, as its development may suggest a need to alter surgical technique.
Adequate brain relaxation is typically achieved with a standard anesthetic including sub-MAC volatile anesthesia, an opioid infusion, mild-to-moderate hyperventilation, and mannitol. In addition, minimizing tumor edema may be accomplished with the administration of dexamethasone.173 Further relaxation can be achieved with discontinuation of the volatile anesthetic and initiation of a propofol infusion. HTS is a reasonable alternative to mannitol, particularly in the setting of anuric renal failure when mannitol is contraindicated. A randomized trial showed that 3% saline and mannitol have equivalent brain relaxation effects, but with the former having less electrolyte and vascular volume sequelae.125 A brain that remains “full” may be the result of venous congestion. This problem can be mitigated with head-up tilt, but is best prevented by positioning the patient so as to minimize excessive rotation or angulation of the neck. The central venous pressure and jugular bulb venous pressure (if available) can be transduced to confirm the absence of a pressure gradient across the neck.
Vascular tumors such as meningioma may benefit from preoperative embolization. Surgical removal of large ones or ones that could not be embolized represents a high risk for significant operative blood loss. Coagulopathy can develop as a consequence of massive transfusion. It is important to perform frequent coagulation studies and administer clotting products and platelets promptly.
Pituitary Surgery
Masses in the region of the sella turcica most commonly are of pituitary origin, although other benign (meningioma, craniopharyngioma) and malignant (germ cell tumor, lymphoma) tumors may occur in this region. These tumors are typically recognized as a result of the neurologic changes they effect as they compress adjacent structures, such as visual changes with impingement of the optic chiasm, or through the systemic effects they exert via a change in hormone secretion.
Although many patients with sellar tumors may undergo surgical resection with an uncomplicated general anesthetic, there are several preoperative considerations that will affect the management of the patient. Patients should undergo a preoperative evaluation of their hormonal function to detect hypersecretion of pituitary hormones, common in pituitary adenomas, as well as panhypopituitarism. The hormones that may be secreted by pituitary tumors include prolactin, growth hormone, corticotropin, and thyroid-stimulating hormone. Patients with excess growth hormone eventually will develop acromegaly. The anesthesiologist should be prepared for a difficult airway, as well as postoperative respiratory complications in the acromegalic patient. Patients with a corticotropin-secreting adenoma will develop Cushing disease. These patients may have a typical “Cushingoid” habitus that may make airway management challenging. In addition, venous access may be difficult, and intraoperative hyperglycemia is likely. Patients with thyroid-stimulating hormone hypersecretion will exhibit signs of hyperthyroidism (e.g., tachycardia, weight loss). These patients should be managed in the preoperative period with antithyroid medications and beta-blockade. Close hemodynamic monitoring during surgery is essential.
Patients with panhypopituitarism will need hormone replacement including cortisol, levothyroxine, and possibly DDAVP (1-desamino-8-D-arginin vasopressin). These medications should be continued in the perioperative period.
Small to medium pituitary tumors can be resected from a transsphenoidal approach. Larger tumors may require a craniotomy. IOM of glucose and electrolytes is essential, particularly if the patient has pre-existing diabetes insipidus, or if the patient develops signs of diabetes insipidus during the surgery. Diabetes insipidus is a common complication of pituitary surgery due to the loss of antidiuretic hormone production. It may be temporary or permanent, and may occur either in the intraoperative or postoperative period. It is initially suspected on the basis of copious urine output, as well as rising serum sodium. A urine specific gravity of <1.005 is confirmatory. Although infusions of intravenous fluids containing free water may mitigate the electrolyte changes, replacement of antidiuretic hormone with DDAVP (0.5 to 1 μg intravenously or subcutaneously) is an effective therapy for diabetes insipidus. Volume replacement therapy may be guided with the use of central venous pressure monitoring as well as the observation of systolic variation in blood pressure.
Arteriovenous Malformations
A cerebral AVM is an abnormal vascular connection between the arterial and the venous circulation. The absence of an intervening capillary bed results in a low-resistance path for blood flow. Patients may present with hemorrhage, seizure, or focal neurologic deficit. Cerebral angiography remains the gold standard for AVM diagnosis. Although preoperative embolization of the AVM is commonly performed, either radiosurgery or an open surgical procedure is typically required subsequent to the embolization to cure the lesion. Although these lesions may be adjacent to vital structures, and an immediate postoperative neurologic examination may be desirable, emergence from anesthesia following resection of an AVM requires particular care. Because of local hemodynamic changes as a result of the AVM, the adjacent vessels must chronically vasodilate to preserve perfusion. When the low-resistance AVM has been occluded or resected, the adjacent vessels are exposed to higher pressures than to which they were previously accustomed. These vessels may not be able to autoregulate appropriately within the normal blood pressure range, and “normal perfusion pressure breakthrough” may occur. This phenomenon is defined by regional hyperemia at a normal systemic blood pressure. Normal perfusion pressure breakthrough may result in vasogenic edema and hemorrhage. It can be minimized with careful blood pressure control; preoperative embolization likely decreases its incidence as well. Following resection of large AVMs or those in the posterior fossa, taking the patient to the ICU in a ventilated and sedated state may be appropriate. Should the decision be made between the surgeon and anesthesiologist to allow emergence and tracheal extubation, aggressive management of blood pressure should be instituted, and coughing should be avoided. Intravenous labetalol and hydralazine may be adequate, but a nicardipine infusion may be most appropriate for blood pressure control. Blood pressure control needs to be conducted using an anticipated and prophylactic approach rather than a reactive one, as the delay in treating hypertension may be detrimental. Intravenous lidocaine can be used to blunt coughing.
Cerebral Aneurysm Surgery and Endovascular Treatment
Cerebral aneurysms are relatively prevalent vascular abnormalities (approximately 5% incidence at autopsy) that arise from congenital weakness of the vessel wall as well as extrinsic influences such as hypertension and cigarette smoking. They are more prevalent in women than in men. Some aneurysms become clinically significant when they rupture, resulting in arterial bleeding into the subarachnoid space. This event typically causes severe headache, and may also cause focal neurologic deficit, lethargy, and coma. For patients who survive their hemorrhage, surgical or endovascular intervention to secure the aneurysm is essential to prevent further hemorrhage. In addition, many patients are incidentally found to have cerebral aneurysms, and they may need intervention to decrease the risk of an initial subarachnoid hemorrhage. Intervention for a cerebral aneurysm may include a craniotomy and surgical clipping, or endovascular coiling.
Anesthetic considerations for cerebral aneurysm surgery are somewhat different in those patients who have experienced a subarachnoid hemorrhage as compared with those who present for elective repair. Patients with aneurysmal subarachnoid hemorrhage are at risk for numerous complications that may affect the anesthetic plan. These include cardiac dysfunction, neurogenic or cardiogenic pulmonary edema, hydrocephalus, as well as further hemorrhage from the aneurysm. This last complication is perhaps the most devastating and requires intensive treatment (see also Chapter 55).
Careful attention to hemodynamics, particularly during stimulating procedures, is essential to avoid recurrent hemorrhage. Laryngoscopy and placement of the head in the Mayfield device are two points at which transient hypertension is likely and the anesthesiologist must be particularly vigilant about maintaining an adequate depth of anesthesia.
Following subarachnoid hemorrhage, cardiac dysfunction, and pulmonary edema commonly resolve over time. The cardiac dysfunction may be severe, resulting in ECG changes, elevated troponin, and even cardiogenic shock. Echocardiography may reveal hypokinesis in a distribution not consistent with an anatomic vascular territory. Unfortunately, the need to secure the aneurysm in a timely fashion may require the anesthesiologist to provide anesthesia despite ongoing cardiac and pulmonary issues. Hemodynamic support with carefully titrated vasopressors may be necessary, recognizing the risk of elevating the blood pressure too much. Hypoxemia can often be managed with increased FIO2 and positive end-expiratory pressure. With the exception of a hemodynamically unstable patient, surgical clipping of a ruptured aneurysm should rarely be postponed.
Once the aneurysm is secured with an aneurysm clip, the risk of recurrent hemorrhage from the aneurysm is removed. Although careful attention to hemodynamics as well as coughing during emergence is still important, the concern of devastating hemorrhage from the aneurysm is diminished.
The patient presenting for an elective aneurysm procedure will typically have good brain conditions, with easily achievable relaxation using mannitol (0.5 to 1 g/kg), mild-to-moderate hyperventilation, and sub-MAC volatile anesthetic in combination with an opioid infusion. Following subarachnoid hemorrhage, brain relaxation may be more difficult to achieve; intravenous anesthesia may be required. Drainage of CSF via a lumbar drain or external ventricular drain can be used at the discretion of the surgeon.
In contrast to aneurysm surgery, endovascular treatment of aneurysms is a minimally invasive procedure performed in the interventional radiology suite. The interventional neuroradiologist or neurosurgeon accesses the aneurysm via an intra-arterial catheter and typically deploys coils into the aneurysm that cause it to thrombose. This technique requires a favorably shaped aneurysm that will retain the coils once they are deployed. Increasingly sophisticated techniques, such as placing a stent in the adjacent vessel and coiling through the stent, have increased the range of aneurysms that are amenable to endovascular therapy.
Despite the less invasive nature of this procedure, it can have equally severe complications as surgery, including further hemorrhage, stroke, and vessel dissection.
Although the procedure is not particularly stimulating, the general anesthetic needs to be performed with great care. Obviously, hypertension with laryngoscopy should be avoided. Furthermore, any patient movement during the procedure can incur devastating consequences because it may result in deployment of coils in a vessel rather than the aneurysm itself. Hyperventilation should be avoided, as it will decrease CBF and make access to the aneurysm more challenging. Heparin is commonly administered during this procedure. It is meant to decrease the risk of thromboembolic complications associated with the intra-arterial catheter. Protamine must be available should arterial rupture and extravasation occur. In addition, prompt transfer to an operating room for neurosurgical intervention should also be possible.
Carotid Surgery
Carotid stenosis is a common cause of transient ischemic attack and ischemic stroke. It is amenable to surgical intervention and endovascular stenting. In older studies, CEA was found to be beneficial in reducing stroke rate in symptomatic patients (ipsilateral transient ischemic attack or nondisabling stroke) with ≥70% internal carotid artery stenosis and, to a lesser extent, in patients with 50% to 69% internal carotid artery stenosis.174,175 In asymptomatic carotid stenosis, the benefit of surgical intervention over medical therapy appears to be somewhat smaller, and it depends on the incidence of perioperative stroke.176,177 In addition, surgery is associated not only with a risk of stroke, but also myocardial infarction, wound infection, and cranial nerve dysfunction. At the time of the NASCET trial,174 medical therapy consisted primarily of daily aspirin. With advances in medical therapy, including more aggressive lipid-lowering drugs as well as other effective antiplatelet agents and better antihypertensive therapy, the margin of benefit of surgery may be even less.178 Appropriate candidate selection for surgery has therefore become extremely important. Preoperative evaluation of the asymptomatic patient depends on assessment of the risk for progression to stroke and weighing that risk against the morbidity of the procedure.
Both general and regional anesthesia may be used for CEA. Regional anesthesia is accomplished with a superficial cervical plexus block, or a combination of superficial and deep block.179,180 This technique allows continuous neurologic assessment during the surgery, which is particularly useful at the time of carotid cross-clamp. However, some patients and surgeons may not be agreeable to this anesthetic technique. General anesthesia with an endotracheal tube is therefore a more common technique for CEA. A multicenter, randomized controlled trial, GALA, involving 3,526 patients, compared general anesthesia versus local anesthesia for carotid surgery, and did not show a significant difference between the two groups regarding quality of life, length of hospital stay, and perioperative complications of stroke, myocardial infarction, and death.181 Both techniques are therefore similarly efficacious.
Patients undergoing carotid surgery are nevertheless at increased risk for perioperative complications, given their high prevalence of coronary artery disease, hypertension, chronic obstructive pulmonary disease, diabetes mellitus, and chronic kidney disease. Continuation of beta-blockers and statins is appropriate for patients who are receiving these medications preoperatively. Blood pressure should be maintained as close to baseline as possible throughout the surgery. Without evidence to support it, some advocate raising the blood pressure during carotid cross-clamp to improve flow through collateral vessels. This practice presupposes that collateralization is marginal and will be helped by the elevation in pressure. Collateral flow may be marginal, but it may also be absent or entirely adequate. In the latter two situations, elevation in blood pressure through the use of phenylephrine will only increase myocardial oxygen demand. However, evidence of hypoperfusion ipsilateral to the cross-clamp is reason to consider blood pressure elevation. The decision to raise blood pressure is facilitated by the concurrent use of CNS monitors.
Several CNS monitors may be used during CEA under general anesthesia. EEG allows for easy detection of a decline in spectral power on the hemisphere ipsilateral to the surgery, which would be concerning for ischemia. NIRS is also promising for its ability to demonstrate relative changes between the ipsilateral and contralateral hemispheres, but it has not come into common use as yet. TCD is particularly attractive, as it allows determination of changes in flow during carotid cross-clamp, and detection of emboli, as well as diagnosis of postoperative hyperemia. The hypoperfusion during cross-clamp can be avoided with a shunt during the surgical procedure, but at the increased risk of embolization. A decrease in flow velocity of up to 60% of baseline is generally well tolerated in the anesthetized patient during this procedure. Should a shunt be needed, the development of microembolic signals can provide feedback to the surgeon if there is a modifiable technique to the surgical procedure. At the end of the endarterectomy, during surgical closure, continued presence of a good flow velocity waveform on TCD provides confirmation of stability of the graft and lack of an intimal flap, or thrombosis. Although each monitor has attractive features, ultimately user familiarity and comfort will determine its utility.182 Postoperatively, sustained elevation of flow velocity exceeding 100% of baseline values is highly suggestive of the development of hyperperfusion syndrome, and should prompt lowering of systemic blood pressure.
Rapid emergence and tracheal extubation at the end of the procedure is desirable because it allows immediate neurologic assessment. Hemodynamic changes can occur in the postoperative period from denervation of the carotid baroreceptor. In addition, headache, obtundation, and/or focal neurologic deficit in the postoperative period should prompt one to consider hyperemia, hemorrhage, or ischemic stroke.
Carotid artery stenting (CAS) may be used to treat carotid stenosis as well. It is an attractive procedure in that it is minimally invasive and can be performed under sedation. However, noninferiority studies have not supported stenting as compared with CEA in symptomatic patients.183,184 Yet the SAPPHIRE trial has indicated that stenting may be a reasonable option in asymptomatic patients with tight stenosis; currently, most centers reserve stenting for patients who are poor surgical candidates.185,186 A clinical trial in 2010, comparing CAS and CEA did not show a difference in the primary outcome of stroke, MI, or death among symptomatic and asymptomatic patients.187 However, the patients who underwent CEA had a lower stroke rate, whereas patients who had CAS had a lower rate of myocardial infarction.
Anesthetic considerations for this procedure are important, even though it is typically performed under sedation. These patients tend to have significant medical comorbidities. Conversion to general anesthesia may incur significant risk. Furthermore, the procedure itself may induce significant hemodynamic changes, most notably bradycardia or asystole during balloon angioplasty of the internal carotid artery. Although pretreatment with atropine may prevent this complication, a brisk tachycardia is generally not desirable in these patients.
Epilepsy Surgery and Awake Craniotomy
Some intracranial neurosurgical procedures are performed on “awake” (i.e., sedated and pain free, with local anesthesia, yet able to respond to verbal or visual command) patients in order to facilitate monitoring of the region of the brain on which the surgeon is operating. These procedures require particular attention on the part of the anesthesiologist to provide patient comfort and safety. Typically these surgeries are for tumors adjacent to eloquent cortex or for resection of an epileptic focus. Frequently the decision to perform the procedure awake has been made by the neurosurgeon prior to the patient meeting the anesthesiologist. It is the role of the anesthesiologist to determine whether the patient is an appropriate candidate for an awake procedure, to coordinate with the neurosurgeon the anesthetic plan, and to support and reassure the patient through the process.
Although the patient with a difficult airway, obstructive sleep apnea, or orthopnea may present a relative contraindication to an awake craniotomy, it is the patient with severe anxiety, claustrophobia, or other psychiatric disorder who may be particularly inappropriate for this type of procedure. On the other hand, patients with neurodegenerative disorders are often elderly and may have respiratory, cardiovascular, and autonomic system compromise. Potential drug interactions and adverse effects from anti-Parkinson’s medications may also occur during anesthesia.188 Patients with dystonia or torticollis may present difficulties in airway management. Patients with psychiatric, epileptic, and chronic pain disorders will present with their own set of challenges and will need special consideration in the management of their medications perioperatively.189–191
Preoperative evaluation should be comprehensive and should include a thorough airway examination. Conversion to a general anesthetic remains a possibility at any point during the procedure. Extensive discussion with the patient regarding the plan is essential to prepare him or her for the experience in the operating room.
Intraoperative Management
Standard anesthesia monitors including an ECG, noninvasive arterial blood pressure, oxygen saturation, and end-tidal CO2 are minimum requirements. Where tight control of blood pressure is required, invasive blood pressure monitoring should be employed. O2 is provided through variable or fixed rate oxygen delivery devices with end-tidal CO2 monitoring capability in order to verify airway patency and track respiratory rate. Attention should be given to the positioning of the patients on the operating table for maximal comfort and cooperativeness. Direct visual and verbal contacts with the patient should be maintained throughout the procedure. The environment should be made quiet, calm, and comfortable with an appropriate room temperature and humidity.
Conscious Sedation (CS) Technique
The goal of sedation is to allow the patient to have a minimally depressed level of consciousness but remain able to maintain his/her own airway and respond to verbal stimulation. Only the administration of supplemental O2 via nasal prongs or cannula, or a facemask is required. Sedation may be given throughout the procedure or can be utilized intermittently for the surgically stimulating part of the procedure. Frequently used drugs for CS include propofol, opioids such as fentanyl or remifentanil, and dexmedetomidine.192–194 Generally, benzodiazepines are avoided.
Propofol is one of the most frequently used drug either alone, or in combination with remifentanil. Propofol can be titrated to provide adequate sedation and a rapid, smooth recovery. However, it can decrease the incidence of seizures. At least 15 to 30 minutes is required for discontinuation of the propofol infusion prior to electrocorticographic recording. Retrospective analysis of an AAA (asleep–awake–asleep) sequence using propofol and remifentanil showed that adequate conditions are obtained in 98% of patients, with a median wake-up time of 9 minutes at the mean infusion rates of 50 μg/kg/min.195–197
There is growing evidence suggesting that dexmedetomidine may be the ideal sedative for awake procedures with low-dose infusion rates (0.3 to 0.6 μg/kg/hr).119,198 Dexmedetomidine is a central-acting α2 agonist that offers sedation and anxiolysis, helps maintain hemodynamic stability (through its central α-agonist activity) and has analgesic properties. Dexmedetomidine also causes minimal respiratory depression and has an anesthetic-sparing effect, even with infusions at the higher end of the dose range.
“Asleep Awake Asleep” (AAA) Technique
The AAA pathway is often the preferred method for epilepsy surgery and tumor resection. This anesthetic plan involves general anesthesia for the skin incision (supplemented by scalp blocks or local anesthetic infiltration), initial craniotomy, and then for the closure in the end, while the patient is allowed to emerge from anesthesia for the middle portion of the surgery in which the surgeon is working around the eloquent areas of the brain. Intraoperative mapping of cortical functions in the awake patient helps to identify the precise location of epileptic focus. During such mapping, the neurosurgeon applies electrical stimulation to the exposed brain cortex of the awake patient, which allows the neurophysiologist to report any sensory, motor, and speech effects as well as any effects on more complex cognitive functions. Such mapping can also be performed during resection of the tumor, thereby allowing for maximal tumor resection while minimizing neurologic deficits (the resection is stopped if symptoms emerge). This gives the patient better postoperative quality of life, better chance of survival.
The asleep portions of the procedure may be performed without an airway, with a laryngeal mask airway (LMA), or with an endotracheal tube in place. For suitable candidates, spontaneous ventilation with propofol anesthesia is an attractive option, as it allows straightforward emergence with minimal coughing, gagging, or straining. In addition, propofol provides a nice anesthetic for these patients because of its low incidence of nausea and vomiting during the awake period. An LMA is a suitable alternative to an endotracheal tube as it can frequently be removed with little movement of the patient as he or she emerges from anesthesia. Topical application of lidocaine to the airway prior to insertion of the LMA supplemented with lidocaine jelly on the LMA may improve patient tolerance during emergence.
An endotracheal tube provides the most secure airway, but it is also the most difficult to remove during the procedure, particularly with the patient’s head secured in rigid fixation. If this pathway is chosen, several options exist to minimize coughing as the patient emerges. Prior to placement of the endotracheal tube, the larynx and trachea may be anesthetized with lidocaine. In addition, the cuff of the endotracheal tube can be filled with 4% lidocaine rather than air. Finally, allowing the patient to emerge on an infusion of low-dose remifentanil or dexmedetomidine may facilitate extubation with little movement.
During the awake portion of the procedure, all sedatives are typically withheld. For particularly stimulating events (e.g., drilling) and in coordination with the surgeon, small boluses of propofol may be given. Antiemetics may be given for nausea and small doses of fentanyl for discomfort.
Following this critical portion of the surgery, the patient may be fully anesthetized for the surgical closure. Initiating a propofol infusion and continuing with spontaneous ventilation is again a good option; otherwise, manipulation of the airway to place an LMA or endotracheal tube will be necessary while avoiding the sterile field.
General Anesthesia (GA)
Patient groups more likely to require GA includes infants and children, patients with learning difficulties, certain seizure or continuous movement disorders, severe pain, claustrophobia, and those patients who are critically ill (e.g., with high ICP).
GA may create several problem areas during surgery. For epilepsy surgery, if intraoperative localization of the epileptic focus is needed in order to provide good conditions for electrocorticography and for motor testing, the influence of anesthetic agents should be kept at a minimum, while concomitantly avoiding long periods of potential awareness on the part of the patient. Specific preoperative preparation should include informing the patients of the possibility that awareness and recall may occur at the time of electrocorticographic recording, but reassuring them that this will be brief and painless. All anesthetics will affect electrocorticography but the use of the shorter-acting anesthetic agents, either inhalation agents and/or intravenous agents will allow for faster decrease in the depth of anesthesia. During the time of recording, all or most of the anesthetic agents are discontinued. Long-term anticonvulsant therapy may lead to increased dosage requirements for opioids and neuromuscular blocking agents. Nitrous oxide should be avoided to prevent complications from a pneumocephalus. Complications that may occur during epilepsy surgery are similar to those for any craniotomy. However, severe bradycardia is a common occurrence with resection of the amygdala–hippocampus.
ANESTHESIA AND TRAUMATIC BRAIN INJURY
Overview of Traumatic Brain Injury
The presence of TBI is the primary determinant in the quality of outcome for patients suffering from trauma.34 Anesthesiologists are involved in the care of these patients in many different settings, including the initial resuscitation in the emergency department, anesthetic management in the operating room, and ongoing care in the ICU (see also Chapter 55).
Primary injury is the result of the initial, mechanical forces on the brain tissue and skull, resulting in skull fracture, brain contusion and/or intracranial hematoma as a result of shearing and compression of neuronal, glial, and vascular tissues. The consequences of the initial TBI include physical disruption of cell membranes and infrastructure, and disturbance of ionic homeostasis secondary to increased membrane permeability. This in turn may lead to astrocytic and neuronal swelling, relative hypoperfusion, perturbation of cellular calcium homeostasis, increased free radical generation and lipid peroxidation, mitochondrial dysfunction, inflammation, apoptosis, and diffuse axonal injury. These mechanisms result in irreversible damage.34 Secondary injury is the progression of the pathologic insults, such as ischemia, reperfusion and hypoxia starting immediately after the initial TBI. Systemic insults such as hypotension (systolic BP <90 mm Hg), hypoxemia (PaO2 <60 mm Hg), hypoglycemia, hyperglycemia, hypocarbia, and hypercarbia are major contributors to secondary injury. The initial approach to patients with TBI should be similar to that of any trauma patient, as outlined in Advanced Trauma Life Support (ATLS) by the American College of Surgeons and followed by limitation of secondary insults. Although delayed brain ischemia appears to be the major common pathway of secondary brain damage, reperfusion hyperemia may also occur.
Airway and breathing are obviously of paramount importance in any critically ill patient but even more so in patients with head injuries, given the sensitivity of the brain to hypoxemia and hypercapnia. Prehospital tracheal intubation of the patient with TBI may be associated with worse outcomes.199–205 If the patient arrives in the emergency department intubated, one must confirm proper placement of the endotracheal tube with a CO2 detector and chest radiograph.200,202–205 If the patient is not intubated, immediate attention should focus on assessing the airway and making preparations for intubation. Patients with TBI usually have several indications for intubation including: Decreased level of consciousness, increased risk of aspiration, as well as concern for hypoxemia and hypercarbia. Sometimes these patients must be intubated and sedated simply to allow further diagnostic studies. SCI occurs in up to 2% to 5% of all major trauma cases and at least 14% of these cases have the potential to have an unstable spine. Techniques to minimize cervical spine (C-spine) movement should be employed during airway management, while considerations should be given to other potential injuries.
Patients with TBI have up to a 10% incidence of an unstable cervical spine injury.206,207 Risk factors include a motor vehicle accident and Glasgow Coma Scale (GCS) score <8. Therefore, all attempts at intubation should include in-line neck stabilization to decrease the chance of worsening a neurologic injury.208 This maneuver may worsen the view of the glottis, making intubation more difficult.209 Therefore, one must always have a backup plan and device in mind when performing an emergency intubation, including but not limited to LMAs and fiberoptic or videolaryngoscope technology. Patients with TBI should generally be intubated orally, as the potential presence of a basilar skull fracture could increase the risk associated with a nasal intubation. A surgical airway remains an appropriate procedure for patients with severe facial trauma and an expected difficult airway.
Minimizing the risk of aspiration of gastric contents during airway procedures is essential. The effectiveness and correct application of cricoid pressure (CP) in this setting has been questioned.210,211 New evidence suggests that the alimentary canal at the level of cricoid ring is the postcricoid hypopharynx and not the esophagus.212 Rice et al.213 define the concept of “cricoid pressure unit” and discredit the logic of ineffectiveness of the CP due to previously reported displacement of the esophagus in relation to the trachea. Incorrectly applied CP may displace cervical fractures, whereas bimanual application may help to reduce this displacement.214,215
Another important consideration is the choice of drugs to facilitate intubation. As discussed previously, hypotension is extremely detrimental to the injured brain; therefore, the choice of drugs must be tailored to each individual patient. Sodium thiopental in a dose of 3 to 6 mg/kg is a useful drug in euvolemic hemodynamically stable patients, but is no longer available in the United States. Through its depressant effect on cerebral metabolism, thiopental decreases CBF, CBV, and ICP,216,217 but may cause profound hypotension. Propofol and etomidate are suitable alternatives, with the latter causing less hypotension at the expense of adrenal suppression. In doses of 1.5-mg/kg lidocaine blunts the effects of laryngoscopy and intubation on ICP with minimal hemodynamic effects. Finally, although controversial, succinylcholine is not contraindicated in patients with TBI, as the effects on ICP are clinically insignificant. Succinylcholine may be the relaxant of choice when intubation needs to be established quickly.218–220 On the other hand, rocuronium is a suitable alternative when no difficulty with the airway or intubation is anticipated. Once the trachea is intubated, the initial ventilation parameters should include 100% O2; arterial CO2 should be maintained in the lower normal range (35 mm Hg), and the subsequent ventilation regimen should be guided by arterial blood gas analysis.
The goal of resuscitation in any trauma patient should be to establish adequate circulation, with oxygenated blood, so that cerebral perfusion may be maintained. Blood pressure and CPP are often low after TBI. Several studies have documented worsened outcome in TBI patients who have experienced episodes of hypotension (SBP < 90 mm Hg) after TBI. Thus, continuous monitoring and optimization of blood pressure and CPP are essential. It is worth noting that there is a lack of information on what the optimal intraoperative hemodynamic goals should be. Cerebral autoregulation may be impaired after TBI. This has two possible consequences: (a) when blood pressure is low, cerebral ischemia may result, (b) in the presence of high blood pressures, cerebral hyperemia and hemorrhage may ensue. The overwhelming evidence of harm from hypotension necessitates restoration of intravascular volume.101 Isotonic crystalloid solutions should be used to accomplish this goal; note that lactated Ringer solution is slightly hypotonic (Table 36-3). The goal is to maintain CPP in the range of 50 to 70 mm Hg, as recommended by the guidelines from the Brain Trauma Foundation in 2007.221 Glucose-containing solution should definitely be avoided. The role of colloids is controversial. According to the Saline versus Albumin Fluid Evaluation (SAFE) study, resuscitation with albumin is associated with higher mortality rate and unfavorable outcome in TBI patients.142 In regards to synthetic colloids, recently published studies indicate that administration of hydroxyethyl starch in patients with severe TBI may be relatively safe, but confirmation is needed.222–224 A multicenter, clinically randomized control trial to determine whether out-of-hospital administration of hypertonic fluids would improve neurologic outcome following severe TBI has been terminated early due to futility. The investigators concluded that initial fluid resuscitation of patients with severe TBI with either HTS/dextran or HTS was not superior to 0.9% saline with respect to 6-month neurologic outcome or survival.225
TABLE 36-3. INTRAVENOUS FLUIDS
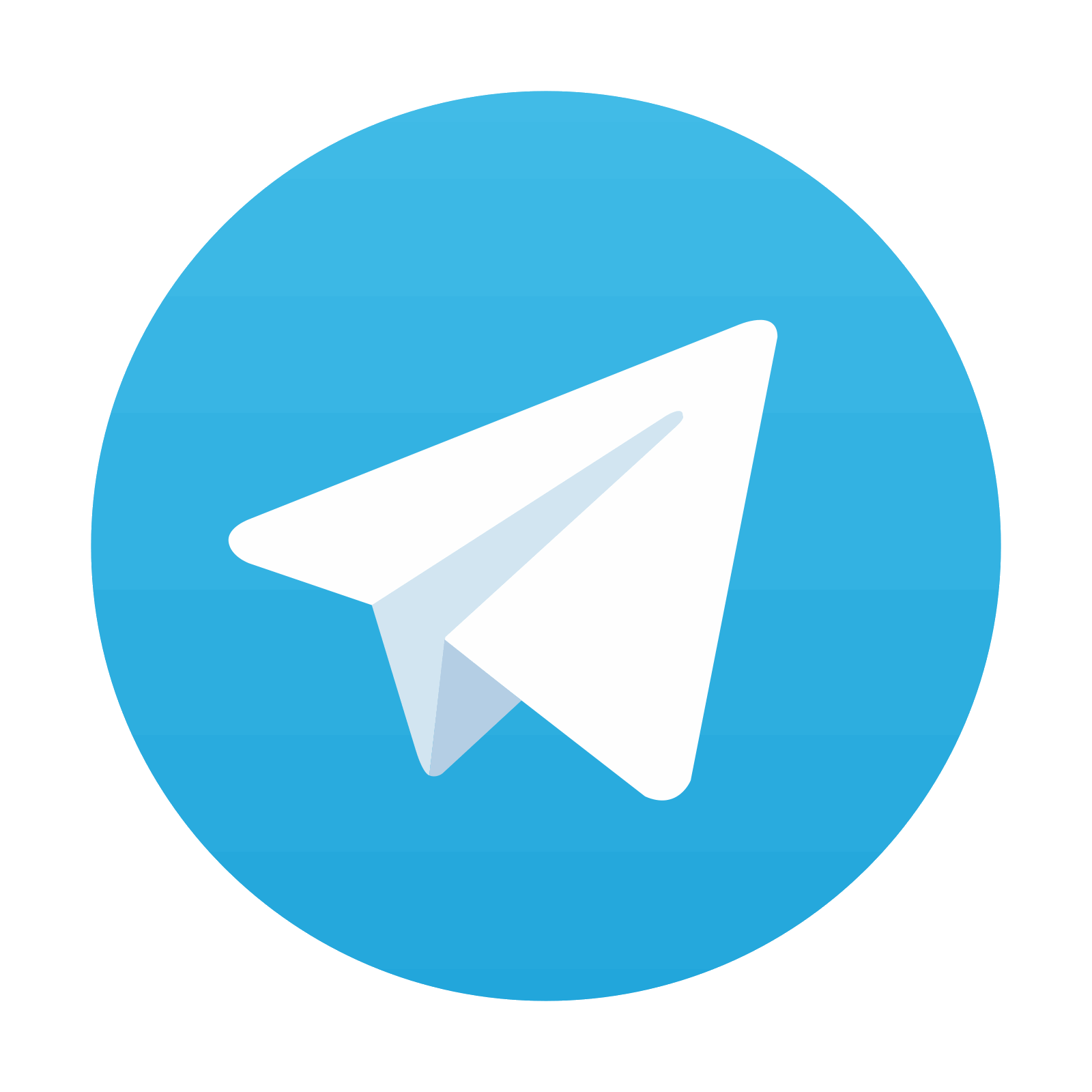
Stay updated, free articles. Join our Telegram channel
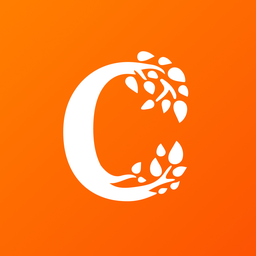
Full access? Get Clinical Tree
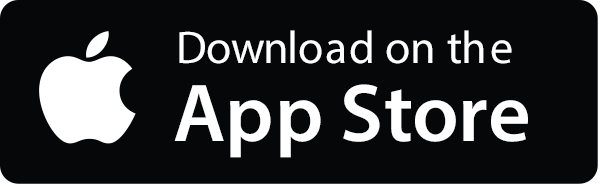
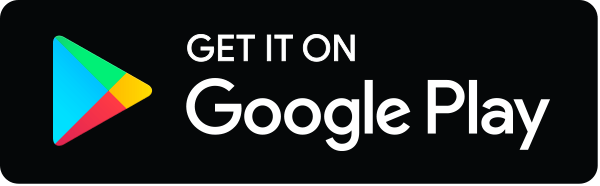