FIGURE 12.1 Illustration depicting the different tendencies of colloid and crystalloid fluids to expand the plasma volume and the interstitial fluid volume. See text for further explanation.
Isotonic Saline
One of the most widely used crystalloid fluids is 0.9% sodium chloride (1), with annual sales of 200 million liters in the United States (data from Baxter Healthcare). This solution has a variety of names, including normal saline, physiologic saline, and isotonic saline, none of which is appropriate (see next). The term used for 0.9% NaCL in this text is isotonic saline, to distinguish it from hypertonic saline (described later).
Normal Saline is Not Normal
The most popular term for 0.9% NaCL is normal saline, but this solution is neither chemically nor physiologically normal. It is not normal chemically because the concentration of a one-normal (1 N) NaCL solution is 58 grams per liter (the combined molecular weights of sodium and chloride), while 0.9% NaCL contains only 9 grams of NaCL per liter. It is not normal physiologically because the composition of 0.9% NaCL differs from the composition of extracellular fluid. This is shown in Table 12.1. When compared to plasma (extracellular fluid), 0.9% NaCL has a higher sodium concentration (154 vs. 140 mEq/L), a much higher chloride concentration (154 vs. 103 mEq/L), a higher osmolality (308 vs. 290 mOsm/L), and a lower pH (5.7 vs. 7.4). These differences can have deleterious effects on fluid and acid-base balance, as described next.
Table 12.1 Comparison of Plasma and Crystalloid Resuscitation Fluids
Volume Effects
The effects of 0.9% NaCL on expanding the plasma volume and interstitial fluid volume are shown in Figure 12.2. Infusion of one liter of 0.9% NaCL adds 275 mL to the plasma volume and 825 mL to the interstitial volume (2). This is the distribution expected of a crystalloid fluid. However, there is one unexpected finding; i.e., the total increase in extracellular volume (1,100 mL) is slightly greater than the infused volume. This is the result of a fluid shift from the intracellular to extracellular fluid, which occurs because 0.9% NaCL is slightly hypertonic in relation to extracellular fluid, as shown in Table 12.1.
INTERSTITIAL EDEMA: Infusions of 0.9% NaCL promote interstitial edema more than crystalloid fluids with a lower sodium content (e.g., Ringer’s lactate, Plasma-Lyte) (3). This is related to the increased sodium load from 0.9% NaCL, which increases the “tonicity” of the interstitial fluid (as just described) and promotes sodium retention by suppressing the renin-angiotensin-aldosterone axis (4). Decreases in renal perfusion have also been observed after infusion of 0.9% NaCL (3), presumably as a result of chloride-mediated renal vasoconstriction. The increase in interstitial edema with 0.9% NaCL can have a negative influence on clinical outcomes (5).
FIGURE 12.2 The effects of selected colloid and crystalloid fluids on the plasma volume and interstitial fluid volume. The infusion volume of each fluid is shown in parentheses. Data from Reference 2.
Acid-Base Effect
Large-volume infusions of 0.9% NaCL produce a metabolic acidosis (6,7), as demonstrated in Figure 12.3. In this clinical study (6), infusion of isotonic saline (0.9% NaCL) at a rate of 30 mL/kg/h was accompanied by a progressive decline in the pH of blood (from 7.41 to 7.28) over two hours, while the pH was unchanged when Ringer’s lactate solution was infused at a similar rate. The saline-induced metabolic acidosis is a hyperchloremic acidosis, and is caused by the high concentration of chloride in 0.9% saline relative to plasma (154 versus 103 mEq/L). The close match between the chloride concentration in Ringer’s lactate solution and plasma (see Table 12.1) explains the lack of a pH effect associated with large-volume infusion of Ringer’s lactate solution.
STRONG ION DIFFERENCE: The influence of crystalloid fluids on acid-base balance can also be explained using the strong ion difference (SID), which is the difference between readily dissociated (strong) cations and anions in extracellular fluid (8). (The SID of plasma is roughly equivalent to the plasma [Na] – plasma [CL] since these are the most prevalent strong ions in extracellular fluid. Plasma HCO3 is not included in the SID because HCO3 is not a strong ion.) The principle of electrical neutrality requires an equal concentration of cations and anions in the extracellular fluid, so the relationship between SID and the ions that dissociate from water (H+ and OH–) can be described as follows:
FIGURE 12.3 The effects of isotonic saline (0.9% NaCL) versus lactated Ringer’s solution on the pH of blood in patients undergoing elective surgery. Total volume infused after 2 hours was 5 to 6 liters for each fluid. Data from Reference 6.
(12.1)
Since the [OH–] is negligible in the physiologic pH range, equation 12.1 can be rewritten as:
(12.2)
According to this relationship, a change in SID must be accompanied by a reciprocal change in [H+] (or a proportional change in pH) to maintain electrical neutrality. The relationship between the SID and pH of plasma is shown in Figure 12.4 (9). Note that the SID and pH change in the same direction (because pH is used instead of [H+]). The normal SID of plasma is 40 mEq/L (as indicated by the dotted line), which is roughly equivalent to the normal plasma [Na+] – [CL–] difference (140 – 103 mEq/L).
The SID of intravenous fluids determines their ability to influence the pH of plasma. The SID of 0.9% NaCL is zero (Na – CL=154 – 154=0) , so infusions of 0.9% NaCL will reduce the SID of plasma and thereby reduce the plasma pH. The SID of Ringer’s lactate fluid is 28 mEq/L (Na + K + Ca – CL=130 + 4 + 3 – 109=28) if all the infused lactate is metabolized. This SID is not far removed from the normal SID of plasma, so Ringer’s lactate infusions will have less of an influence on plasma pH than 0.9% NaCL.
FIGURE 12.4 The relationship between the strong ion difference (SID) and the pH of extracellular fluid (plasma). The normal SID of plasma is about 40 mEq/L. The SID of a crystalloid fluid relative to plasma determines the tendency of the fluid to influence acid-base status. See text for further explanation. Graph redrawn from Reference 9.
Ringer’s Fluids
Sydney Ringer, a British physician who studied the contraction of isolated frog hearts, introduced a sodium chloride solution in 1880 that contained calcium and potassium to promote cardiac contraction and cell viability (10). This solution is shown as Ringer’s injection in Table 12.1, and is essentially 0.9% NaCL with potassium and ionized calcium added.
Ringer’s Lactate
In the early 1930’s, an American pediatrician named Alexis Hartmann added sodium lactate to Ringer’s solution to provide a buffer for the treatment of metabolic acidosis (10). This solution was originally called Hartmann’s solution, and is now known as Ringer’s lactate solution. The composition of this solution is shown in Table 12.1. The sodium concentration in Ringer’s lactate is reduced to compensate for the sodium released from sodium lactate, and the chloride concentration is reduced to compensate for the negatively-charged lactate molecule; both changes result in an electrically neutral salt solution.
Ringer’s Acetate
Because of concerns that large-volume infusions of Ringer’s lactate solution could increase plasma lactate levels in patients with impaired lactate clearance (e.g., from liver disease), the lactate buffer was replaced by acetate to create Ringer’s acetate solution. Acetate is metabolized in muscle rather than liver (10), which makes Ringer’s acetate a reasonable alternative to Ringer’s lactate in patients with liver failure. (The influence of Ringer’s lactate on serum lactate levels is described below). As shown in Table 12.1, the composition of Ringer’s acetate and Ringer’s lactate solutions is almost identical with the exception of the added buffer.
Advantages & Disadvantages
The principal advantage of Ringer’s lactate and Ringer’s acetate over isotonic saline (0.9% NaCL) is the lack of a significant effect on acid-base balance. The principal disadvantage of Ringer’s solutions is the calcium content; i.e., the ionized calcium in Ringer’s solutions can bind to the citrated anticoagulant in stored RBCs and promote clot formation. For this reason, Ringer’s solutions are contraindicated as diluent fluids for the transfusion of erythrocyte concentrates (packed red blood cells) (11). However, clot formation does not occur if the volume of Ringer’s solution does not exceed 50% of the volume of packed RBCs (12).
LACTATE CONSIDERATIONS: As mentioned above, the lactate content in Ringer’s lactate solution (28 mM/L) creates concern about the risk of spurious hyperlactatemia with large-volume infusions of the fluid. In healthy subjects, infusion of one liter of lactated Ringer’s over one hour does not raise serum lactate levels (8). In critically ill patients, who may have impaired lactate clearance from circulatory shock or hepatic insufficiency, the impact of lactated Ringer’s infusions on serum lactate levels is not known. However, if lactate clearance is zero, the addition of one liter of lactated Ringer’s to a blood volume of 5 liters (which would require infusion of 3–4 liters of fluid) would raise the serum lactate level by 4.6 mM/L (13). Therefore, lactated Ringer’s infusions are unlikely to have a considerable impact on serum lactate levels unless large volumes are infused in patients with virtually no capacity for clearing lactate from the bloodstream.
Blood samples obtained from intravenous catheters that are being used for lactated Ringer’s infusions can yield spuriously high serum lactate determinations (14). Therefore, in patients receiving lactated Ringer’s infusions, blood samples for lactate measurements should be obtained from sites other than the infusion catheter.
Other Balanced Salt Solutions
Two of the crystalloid fluids in Table 12.1 (i.e., Normosol and Plasma-Lyte) contain magnesium instead of calcium, and contain both acetate and gluconate buffers to achieve a pH of 7.4 These fluids are not as popular as isotonic saline or Ringer’s lactate, but the absence of calcium makes them suitable as diluents for RBC transfusions, and Plasma-Lyte has shown less of a tendency to promote interstitial edema when compared with isotonic saline (3,5).
Hypertonic Saline
Hypertonic saline solutions like 7.5% NaCL (which has an osmolality 8–9 times greater than plasma) are much more effective at expanding the extracellular volume than isotonic crystalloid fluids. This is demonstrated in Figure 12.2, which shows that infusion of 250 mL of 7.5% NaCL results in a 1,235 mL increase in extracellular fluid, which is about 5 times greater than the infusion volume. (The added volume comes from the intracellular fluid.) Animal studies have shown that hypertonic saline is effective for limited-volume resuscitation of hemorrhagic shock. This is demonstrated in Figure 12.5, which shows that hypertonic saline can restore and maintain cardiac output with 1/5 the volume required with isotonic saline (15).
FIGURE 12.5 A comparison of the cumulative volume of three intravenous fluids needed to maintain a normal rate of aortic blood flow in an animal model of hemorrhagic shock. Data from Reference 15.
Observations like the one in Figure 12.5 suggested that hypertonic saline would be well suited for situations where small volumes of resuscitation fluid are advantageous; e.g., the prehospital resuscitation of trauma victims, particularly those with traumatic brain injury (16). Unfortunately, the accumulated evidence shows no apparent survival benefit from hypertonic saline compared to isotonic crystalloids for the management of traumatic shock (17) or traumatic brain injury (18). The addition of 6% dextran-70 to hypertonic saline to make a hyperoncotic-hypertonic fluid has not improved the results (16.18,19). As a result, hypertonic resuscitation is currently in the graveyard of resuscitation strategies.
5% DEXTROSE SOLUTIONS
The once-popular use of 5% dextrose solutions (D5 solutions) has fallen out of favor, as explained in this section.
Protein-Sparing Effect
Prior to the standard use of enteral tube feedings and total parenteral nutrition (TPN), 5% dextrose solutions were used to provide calories in patients who were unable to eat. Dextrose provides 3.4 kilocalories (kcal) per gram when fully metabolized, so a 5% dextrose solution (50 grams dextrose per liter) provides 170 kcal per liter. Infusion of 3 liters of a D5 solution daily (125 mL/min) provides 3 x 170=510 kcal/day, which is enough nonprotein calories to limit the breakdown of endogenous proteins to provide calories (i.e., protein-sparing effect). This is no longer necessary, as most patients can tolerate enteral tube feedings, and those who cannot will receive TPN.
Volume Effects
The addition of dextrose to intravenous fluids increases osmolality (50 g of dextrose adds 278 mOsm/L to an intravenous fluid). For a 5% dextrose-in-water solution (D5W), the added dextrose brings the osmolality close to that of plasma. However, since the dextrose is taken up by cells and metabolized, this osmolality effect rapidly wanes, and the added water then moves into cells. This is shown in Figure 12.2. The infusion of one liter of D5W results in an increase in extracellular fluid (plasma plus interstitial fluid) of about 350 mL, which means the remaining 650 mL (two-thirds of the infused volume) has moved intracellularly. Therefore, the predominant effect of D5W is cellular swelling.
An effect opposite to that of D5W can occur when dextrose is added to 0.9% NaCL. As described previously, the osmolality of 0.9% NaCL is slightly higher than extracellular fluid (308 vs. 290 mOsm/L), and this results in some movement of water out of cells. When 50 grams of dextrose is added to make D5-normal saline, the osmolality of the fluid increases to 560 mOsm/L, which is almost twice the normal osmolality of the extracellular fluid. If glucose utilization is impaired (as is common in critically ill patients), large-volume infusions of D5W can result in cellular dehydration.
Enhanced Lactate Production
In healthy subjects, only 5% of an infused glucose load will result in lactate formation, but in critically ill patients with tissue hypoperfusion, as much as 85% of glucose metabolism is diverted to lactate production (20). This latter effect is demonstrated in Figure 12.6. In this case, tissue hypoperfusion was induced by aortic clamping during abdominal aortic aneurysm surgery (21). Patients received intraoperative fluids to maintain normal cardiac filling pressures using either a Ringer’s solution or a 5% dextrose solution. When the dextrose-containing fluid was infused, the serum lactate levels began to rise after the aorta was cross-clamped, and the increase in circulating lactate levels persisted throughout the remainder of the surgery. These results indicate that, when circulatory flow is compromised, infusion of 5% dextrose solutions can result in lactic acid production and significant elevations of serum lactate.
Hyperglycemia
About 20% of patients admitted to ICUs are diabetic (22), and as many as 90% of patients will develop hyperglycemia at some time during their ICU stay (23). Hyperglycemia has several deleterious effects in critically ill patients, including immune suppression (22), increased risk of infection (23), aggravation of ischemic brain injury (24), and increased mortality, particularly following cardiac surgery (23). Because of the association between hyperglycemia and increased morbidity and mortality, blood glucose levels are typically not allowed to remain above 180 mg/dL in ICU patients (25).
FIGURE 12.6 The effect of intravenous fluid therapy with and without dextrose on blood lactate levels in patients undergoing abdominal aortic aneurysm repair. Each point represents the mean lactate level in 10 study patients. The average infusion volume for each fluid is indicated in parentheses. Data from Reference 21.
Considering the high risk of hyperglycemia in ICU patients, and the numerous adverse consequences of hyperglycemia, infusion of dextrose-containing fluids should be avoided whenever possible. In fact, considering the potential for harm, it seems that the routine use of 5% dextrose solutions should be abandoned in critically ill patients.
COLLOID FLUIDS
In chemical terms, a colloidal solution is a particulate solution with particles that do not dissolve completely. (These solutions are also called suspensions). In clinical terms, a colloid fluid is a saline solution with large solute molecules that do not pass readily from plasma to interstitial fluid. The retained molecules in a colloid fluid create an osmotic force called the colloid osmotic pressure or oncotic pressure that holds water in the vascular compartment, as reviewed next.
Capillary Fluid Exchange
The direction and rate of fluid exchange (Q) between capillary blood and interstitial fluid is determined, in part, by the balance between the hydrostatic pressure in the capillaries (Pc), which promotes the movement of fluid out of capillaries, and the colloid osmotic pressure of plasma (COP), which favors the movement of fluid into capillaries.
(12.3)
In the supine position, the normal Pc averages about 20 mm Hg (30 mm Hg at the arterial end of the capillaries and 10 mm Hg at the venous end of the capillaries); the normal COP of plasma is about 28 mm Hg (26), so the net forces normally favor the movement of fluid into capillaries (which preserves the plasma volume). About 80% of the plasma COP is due to the albumin fraction of plasma proteins (26), so a reduction in the plasma albumin concentration (hypoalbuminemia) favors the movement of fluid out of the capillaries and promotes interstitial edema.
Resuscitation Fluids
The volume distribution of colloid and crystalloid fluids can be explained by their influence on the plasma COP. Crystalloid fluids re-duce the plasma COP (dilutional effect), which favors the movement of these fluids out of the bloodstream. Colloid fluids can preserve the normal COP (iso-oncotic fluids), which holds these fluids in the bloodstream, or they can increase the plasma COP (hyperoncotic colloid fluids), which pulls interstitial fluid into the bloodstream.
Volume Effects
The volume distribution of a (nearly iso-oncotic) colloid fluid is demonstrated in Figure 12.2. The colloid fluid in this case is a 5% albumin solution, which has a COP of 20 mm Hg. Infusion of one liter of this solution results in a 700 mL increment in the plasma volume and a 300 mL increment in the interstitial fluid volume. When compared with the increment in plasma volume after one liter of 0.9% NaCL (275 mL), the colloid fluid is about 3 times more effective in expanding the plasma volume than the crystalloid fluid. Most observations indicate that, in equivalent volumes, colloid fluids are at least three times more effective than crystalloid fluids for expanding the plasma volume (2, 27–29).
As expected from Figure 12.2, colloid fluids promote cardiac output at much lower infusate volumes than required with crystalloid fluids. This is demonstrated in Figure 12.5 (described earlier), which shows that restoring the cardiac output in hemorrhagic shock requires five times more volume when a crystalloid fluid (isotonic saline) is infused instead of a colloid fluid (dextran-70).
Colloid Fluid Comparisons
As mentioned earlier, the ability of a colloid fluid to augment the plasma volume is determined by the COP of the fluid relative to the plasma COP. This is demonstrated in Table 12.2, which includes the commonly used colloid fluids in the United States, along with the oncotic pressure (COP) of each fluid and the increment in plasma volume produced by a given infusate volume. Note that the higher the COP of the fluid, the greater the increment in plasma volume relative to the infusate volume. Fluids with a COP of 20–30 mm Hg are considered to be iso-oncotic fluids (i.e., fluid COP equivalent to plasma COP); these fluids produce increments in plasma volume that are roughly equivalent to the infusate volume (range = 70–130% of infusate volume). Colloid fluids with a COP >30 mm Hg are hyperoncotic fluids (fluid COP >plasma COP); these fluids produce increments in plasma volume that are usually greater than the infusate volume. This is most apparent with 25% albumin, which has a COP of 70 mm Hg, and produces an increment in plasma volume that is 3 to 4 times greater than the infusate volume.
Table 12.2 Characteristics of Individual Colloid Fluids
Albumin Solutions
Albumin is a versatile plasma protein with several functions. It is the principal determinant of plasma COP (26), the principal transport protein in blood (see Table 12.3), has significant antioxidant activity (30), and helps maintain the fluidity of blood by inhibiting platelet aggregation (31). As much as 2/3 of the albumin in the body is located outside blood vessels (32); the role of the extravascular albumin pool is unclear.
Features
Albumin solutions are heat-treated preparations of human serum albumin that are available as a 5% solution (50 g/L) and a 25% solution (250 g/L) in 0.9% NaCL. The 5% albumin solution is usually given in aliquots of 250 mL; the colloid osmotic pressure is 20 mm Hg, and the plasma volume increment averages 100% of the infused volume. The volume effect begins to dissipate at 6 hours, and can be lost after 12 hours (2,27).
Table 12.3 Substances Transported by Albumin
The 25% albumin solution is a hyperoncotic fluid with a colloid osmotic pressure of 70 mm Hg (more than twice that of plasma). It is given in aliquots of 5 0–100 mL, and the plasma volume increment is 3 to 4 times the infusate volume. The effect is produced by fluid shifts from the interstitial space, so interstitial fluid volume decreases as plasma volume increases. Because it does not replace lost volume, but instead shifts fluid from one compartment to another, 25% albumin should not be used for volume resuscitation in patients with blood loss. This fluid should be reserved for instances where hypovolemia is the result of hypoalbuminemia, which promotes fluid shifts from plasma to interstitial fluid.
Safety
Albumin’s reputation was sullied in 1998 when a clinical review suggested that one of every 17 patients who received albumin infusions died as a result of the fluid (33). This has been refuted in subsequent studies showing that albumin poses no greater risk of death than other volume expanders (34,35). The consensus opinion at the present time is that 5% albumin is safe to use as a resuscitation fluid (32), except possibly in traumatic head injury, where one large study has shown a higher mortality rate in patients who received albumin instead of isotonic saline (36). Hyperoncotic (25%) albumin has been associated with an increased risk of renal injury and death in patients with circulatory shock (37), which is similar to the renal injury reported with other hyperoncotic colloid fluids (see next).
Hydroxyethyl Starch
Hydroxyethyl starch (HES) is a chemically modified polysaccharide composed of long chains of branched glucose polymers substituted periodically by hydroxyl radicals (OH), which resist enzymatic degradation. HES elimination involves hydrolysis by amylase enzymes in the bloodstream, which cleave the parent molecule until it is small enough to be cleared by the kidneys. The following is a summary of the important features of HES preparations (32,38).
Features
MOLECULAR WEIGHT: HES preparations have different molecular weights, and are classified as high MW (450 kilodaltons or kD), medium MW (200 kD), and low MW (70 kD). High MW preparations have a prolonged duration of action because amylase cleavage results in progressively smaller molecules that are osmotically active. When the cleavage products reach a molecular weight of 50 kD, they can be cleared by the kidneys (32).
MOLAR SUBSTITUTION RATIO: HES preparations are also classified by the ratio of hydroxyl radical substitutions per glucose polymer (OH/glucose), which is called the molar substitution ratio and ranges from zero to one (32). Since hydroxyl radicals resist enzymatic degradation, higher OH/glucose ratios are associated with prolonged activity. Higher molar substitution ratios increase the risk of HES-associated coagulopathy (see later).
INDIVIDUAL PREPARATIONS: Individual HES preparations are described by their concentration, MW, and molar substitution ratio, as shown in Table 12.4. Most preparations are available as 6% solutions in 0.9% NaCL. The prefix of the HES preparation indicates the molar substitution ratio (e.g., pentastarch = 0.5, tetrastarch = 0.4). Hetastarch is the most commonly used HES preparation in the United States, and has a high MW(450 kD) and a high molar substitution ratio (0.7). Tetrastarch is the most recent HES preparation introduced for use in the United States, and has the lowest MW (130 kD) and the lowest molar substitution ratio (0.4). Tetrastarch is available as Voluven (Hospira).
Volume Effects
The performance of 6% HES solutions as plasma volume expanders is very similar to 5% albumin. The oncotic pressure is higher than 5% albumin, and the increment in plasma volume can be higher as well (see Table 12.2). The effect on plasma volume can last up to 24 hours with high MW preparations such as hetastarch (38). The duration of action of the lower MW preparations is at least 6 hours, but effects begin to dissipate within one hour (4).
Table 12.4 Characteristics of Hydroxyethylstarch Preparations
Altered Hemostasis
HES can impair hemostasis by inhibition of Factor VII and von Wille-brand factor, and impaired platelet adhesiveness (32,39). This effect was originally attributed to high MW preparations, but high (OH/glucose) ratios are now considered more important in determining the risk of altered hemostasis (32). Clinically significant coagulopathies are uncommon unless large volumes of HES are infused (e.g., >50 mL/kg for tetra-starch) (28).
Nephrotoxicity
Several studies have shown an association between HES infusions and an increased risk of renal injury and death; this association has been reported with hetastarch (40), pentastarch (41), and tetrastarch (42). The colloid osmotic pressure of HES preparations (30 mm Hg for hetastarch and 36 mm Hg for tetrastarch) has been implicated in renal injury, although the precise mechanism is not clear. HES-associated renal injury has been reported mostly in patients with life-threatening conditions such as severe sepsis and circulatory shock (32,41,42). In patients who are less severely ill, there is no association between HES and renal injury (32), and some studies show a favorable responses to HES in such patients (43).
Hyperamylasemia
The amylase enzymes involved in the hydrolysis of HES attach to the HES molecules, and this reduces amylase clearance by the kidneys. This can result is an increase in serum amylase levels to 2–3 times above normal (38,44). Levels usually return to normal within one week after HES is discontinued. Serum lipase levels are unaffected by HES infusions (44).
The Dextrans
The dextrans are glucose polymers produced by a bacterium (Leucono-stoc) incubated in a sucrose medium. First introduced in the 1940s, these colloids are not popular (at least in the United States) because of the perceived risk of adverse reactions. The two most common dextran preparations are 10% dextran-40 and 6% dextran-70, each preparation using 0.9% NaCL as a diluent. The features of 10% dextran-40 are shown in Table 12.2.
Features
Both dextran preparations have a colloid osmotic pressure of 40 mm Hg, and cause a greater increase in plasma volume than either 5% albumin or 6% hetastarch (see Table 12.2). Dextran-70 may be preferred because the duration of action (12 hours) is longer than that of dextran-40 (6 hours) (27).
Disadvantages
1. Dextrans produce a dose-related bleeding tendency that involves impaired platelet aggregation, decreased levels of Factor VIII and von Willebrand factor, and enhanced fibrinolysis (39,44). The hemostatic defects are minimized by limiting the daily dextran dose to 20 mL/kg.
2. Dextrans coat the surface of red blood cells and can interfere with the ability to cross-match blood. Red cell preparations must be washed to eliminate this problem. Dextrans also increase the erythrocyte sedimentation rate as a result of their interactions with red blood cells (44).
3. Dextrans have been associated with an osmotically-mediated renal injury similar to that observed with HES preparations (44,45). However, this complication occurs only rarely with dextran infusions. Anaphylactic reactions, once common with dextrans, are now reported in only .03% of infusions (44).
COLLOID–CRYSTALLOID CONUNDRUM
There is a longstanding debate concerning the type of fluid that is most appropriate for volume resuscitation, and each type of fluid has its loyalists who passionately defend the merits of their chosen fluid. The following is a brief description of the issues involved in this debate, and a suggested compromise.
Early Focus on Crystalloids
Early studies of acute blood loss in the 1960s showed that hemorrhagic shock was associated with an interstitial fluid deficit, partly as a result of a fluid shift from the interstitial fluid into the bloodstream (46). In an animal model of hemorrhagic shock, replacement of the shed blood was almost universally fatal, while survival improved significantly if Ringer’s lactate fluid was added to the replacement of shed blood (47). These results were interpreted as indicating that replacement of the interstitial fluid deficit (with Ringer’s lactate) was the critical factor in the successful resuscitation of hemorrhagic shock. This led to the popularity of crystalloid fluids for the resuscitation of blood loss. Therefore, crystalloid fluids were popularized for volume resuscitation because of their ability to resuscitate the interstitial volume, not the plasma volume.
More Recent Concerns
Since those early studies, the importance of promoting cardiac output and systemic O2 delivery have emerged as the primary focus of volume resuscitation. To this end, colloid fluids have proven superior to crystalloid fluids, as demonstrated in Figure 11.6 (Chapter 11). Despite this superiority, crystalloid fluids remain the popular choice for volume resuscitation (at least in the United States). The principal argument in favor of crystalloid resuscitation is the lack of proven survival benefit with colloid resuscitation (48,49), and the lower cost of crystalloid fluids (see Table 12.5). The problem with crystalloid resuscitation is the relatively large volumes needed to expand the plasma volume (at least 3 times greater than the volume of colloid fluids), which promotes edema formation and a positive fluid balance, both of which are associated with increased morbidity and mortality in critically ill patients (5,50).
Table 12.5 Relative Cost of Intravenous Fluids
A Problem-Based Approach
The colloid-crystalloid controversy is fueled by the premise that one type of fluid is optimal in all cases of hypovolemia. This seems unreasonable, since no single resuscitation fluid will perform optimally in all conditions associated with hypovolemia. The following are some examples of hypovolemia where different resuscitation fluids would be most effective.
1. In cases of life-threatening hypovolemia from blood loss (where a prompt increase in plasma volume is necessary), an iso-oncotic colloid fluid (e.g., 5% albumin) would be most effective.
2. In cases of hypovolemia secondary to dehydration (where there is a uniform loss of extracellular fluid), a crystalloid fluid (e.g., Ringer’s lactate) is appropriate.
3. In cases of hypovolemia where hypoalbuminemia is implicated (causing fluid shifts from plasma to interstitial fluid) a hyperoncotic colloid fluid (e.g., 25% albumin) is an appropriate choice.
As demonstrated in these examples, tailoring the type of resuscitation fluid to the specific cause and severity of hypovolemia is a more reasoned approach than using the same type of fluid for all cases of hypovolemia. Thus, to apply Sir Henry Tizard’s introductory quote to resuscitation fluids, one could say that the secret to selecting the appropriate resuscitation fluid is to ask the question—what is the cause and severity of the hypovolemia in this patient?
A FINAL WORD
The following information in this chapters deserves emphasis:
1. Normal saline (0.9% NaCL) is not normal, either chemically or physiologically, and infusions of this fluid often results in a metabolic acidosis. This does not occur with Ringer’s lactate or Ringer’s acetate solutions.
2. Isotonic crystalloid fluids expand the interstitial fluid volume more than the plasma volume, and large-volume infusion of crystalloid fluids can lead to troublesome edema formation.
3. Colloid fluids are superior to crystalloid fluids for expanding the plasma volume.
4. Hyperoncotic colloid fluids, particularly the hydroxyethyl starches, are associated with an increased risk of renal injury in patients with acute, life-threatening conditions (e.g., severe sepsis and septic shock). This complication is not usually observed in less severely ill patients (e.g., postoperative patients).
5. The colloid-crystalloid debate is misguided because there is no single resuscitation fluid that is optimal for all cases of hypovolemia.
REFERENCES
Crystalloid Fluids
1. Awad S, Allison S, Lobo DN. The history of 0.9% saline. Clin Nutr 2008; 27:179–188.
2. Imm A, Carlson RW. Fluid resuscitation in circulatory shock. Crit Care Clin 1993; 9:313–333.
3. Chowdhury AH, Cox EF, Francis ST, Lobo DN. A randomized, controlled, double-blind crossover study on the effects of 2-L infusions of 0.9% saline and Plasma-Lyte 148 on renal blood flow and renal cortical tissue perfusion in healthy volunteers. Ann Surg 2012; 256:18–24.
4. Lobo DN, Stanga Z, Aloysius MM, et al. Effect of volume loading 1 liter intravenous infusions of 0.9% NaCL, 4% succinated gelatine (Gelofusine), and hydroxyethyl starch (Voluven) on blood volume and endocrine responses: a randomized three-way crossover study in healthy volunteers. Crit Care Med 2010; 38:464–470.
5. Shaw AD, Bagshaw SM, Goldstein SL, et al. Major complications, mortality, and resource utilization after open abdominal surgery: 0.9% saline compared to Plasma-Lyte. Ann Surg 2012; 255:821–829.
6. Scheingraber S, Rehm M, Schmisch C, Finsterer U. Rapid saline infusion produces hyperchloremic acidosis in patients undergoing gynecologic surgery. Anesthesiology 1999; 90:1265–1270.
7. Prough DS, Bidani A. Hyperchloremic metabolic acidosis is a predictable consequence of intraoperative infusion of 0.9% saline. Anesthesiology 1999; 90:1247–1249.
8. Stewart PA. Modern quantitative acid-base chemistry. Can J Physiol Pharmacol 1983; 61:1444–1461.
9. Kellum JA, Elbers PWG, eds. Stewart’s Textbook of Acid Base, 2nd ed. Amsterdam: Acidbase.org, 2009, pg 140.
10. Griffith CA. The family of Ringer’s solutions. J Natl Intravenous Ther Assoc 1986; 9:480–483.
11. American Association of Blood Banks Technical Manual. 10th ed. Arlington, VA: American Association of Blood Banks, 1990:368.
12. King WH, Patten ED, Bee DE. An in vitro evaluation of ionized calcium levels and clotting in red blood cells diluted with lactated Ringer’s solution. Anesthesiology 1988; 68:115–121.
13. Didwania A, Miller J, Kassel; D, et al. Effect of intravenous lactated Ringer’s solution infusion on the circulating lactate concentration: Part 3. Result of a prospective, randomized, double-blind, placebo-controlled trial. Crit Care Med 1997; 25:1851–1854.
14. Jackson EV Jr, Wiese J, Sigal B, et al. Effects of crystalloid solutions on circulating lactate concentrations. Part 1. Implications for the proper handling of blood specimens obtained from critically ill patients. Crit Care Med 1997; 25:1840–1846.
15. Chiara O, Pelosi P, Brazzi L, et al. Resuscitation from hemorrhagic shock: Experimental model comparing normal saline, dextran, and hypertonic saline solutions. Crit Care Med 2003; 31:1915–1922.
16. Patanwala AE, Amini A, Erstad BL. Use of hypertonic saline injection in trauma. Am J Health Sys Pharm 2010; 67:1920–1928.
17. Bunn F, Roberts I, Tasker R, et al. Hypertonic versus near isotonic crystalloid for fluid resuscitation in critically ill patients. Cochrane Database Syst Rev 2004; 3:CD002045.
18. Bulger EM, May S, Brasel KJ, et al. Out-of-hospital hypertonic resuscitation following severe traumatic brain injury. JAMA 2010; 304:1455–1464.
19. Santy HP, Alam HB. Fluid resuscitation: past, present, and future. Shock 2010; 33:229–241.
5% Dextrose Solutions
20. Gunther B, Jauch W, Hartl W, et al. Low-dose glucose infusion in patients who have undergone surgery. Arch Surg 1987; 122:765–771.
21. DeGoute CS, Ray MJ, Manchon M, et al. Intraoperative glucose infusion and blood lactate: endocrine and metabolic relationships during abdominal aortic surgery. Anesthesiology 1989; 71;355–361.
22. Turina M, Fry D, Polk HC, Jr. Acute hyperglycemia and the innate immune system: Clinical, cellular, and molecular aspects. Crit Care Med 2005; 33:1624–1633.
23. Van Den Berghe G, Wouters P, Weekers F, et al. Intensive insulin therapy in critically ill patients. New Engl J Med 2001;345:1359–1367.
24. Sieber FE, Traystman RJ. Special issues: glucose and the brain. Crit Care Med 1992; 20:104–114.
25. Kavanagh BP, McCowen KC. Glycemic control in the ICU. N Engl J Med 2010; 363:25402546.
Colloid Fluids
26. Guyton AC, Hall JE. Textbook of Medical Physiology. 10th ed., Philadelphia: W.B. Saunders, Co, 2000, pp. 169–170.
27. Griffel MI, Kaufman BS. Pharmacology of colloids and crystalloids. Crit Care Clin 1992; 8:235–254.
28. Kaminski MV, Haase TJ. Albumin and colloid osmotic pressure: implications for fluid resuscitation. Crit Care Clin 1992; 8:311–322.
29. Sutin KM, Ruskin KJ, Kaufman BS. Intravenous fluid therapy in neurologic injury. Crit Care Clin 1992; 8:367–408.
30. Halliwell B. Albumin—an important extracellular antioxidant? Biochem Pharmacol 1988; 37:569–571.
31. Soni N, Margarson M. Albumin, where are we now? Curr Anesthes & Crit Care 2004; 15:61–68.
32. Muller M, Lefrant J-Y. Metabolic effects of plasma expanders. Transfusion Alter Transfusion Med 2010; 11:10–21.
33. Cochrane injuries Group Albumin Reviewers: Human albumin administration in critically ill patients: Systematic review of randomized, controlled trials. Br Med J 1998; 317:235–240.
34. Wilkes MN, Navickis RJ. Patient survival after human albumin administration: A meta-analysis of randomized, controlled trials. Ann Intern Med 2001; 135:149–164.
35. SAFE Study Investigators. A comparison of albumin and saline for fluid resuscitation in the Intensive Care Unit. N Engl J Med 2004; 350:2247–2256.
36. The SAFE Study Investigators. Saline or albumin for fluid resuscitation in patients with severe head injury. N Engl J Med 2007; 357:874–884.
37. Schortgen F, Girou E, Deve N, et al. The risk associated with hyperoncotic colloids in patients with shock. Intensive Care Med 2008; 34:2157–2168.
38. Treib J, Baron JF, Grauer MT, Strauss RG. An international view of hydroxyethyl starches. Intensive Care Med 1999; 25:258–268.
39. de Jonge E, Levi M. Effects of different plasma substitutes on blood coagulation: A comparative review. Crit Care Med 2001; 29:1261–1267.
40. Lissauer ME, Chi A, Kramer ME. et al. Association of 6% hetastarch resuscitation with adverse outcomes in critically ill trauma patients. Am J Surg 2011; 202:53–58.
41. Brunkhorst FM, Engel C, Bloos F, et al. Intensive insulin therapy and pentastarch resuscitation in severe sepsis. N Engl J Med 2008; 358:125–139.
42. Perner A, Haase N, Guttormsen AB, et al. Hydroxyethyl starch 130/0.4 versus Ringer’s acetate in severe sepsis. N Engl J Med 2012; 367:124–134.
43. Magder S, Potter BJ, De Varennes B, et al. Fluids after cardiac surgery: A pilot study of the use of colloids versus crystalloids. Crit Care Med 2010; 38:2117–2124.
44. Nearman HS, Herman ML. Toxic effects of colloids in the intensive care unit. Crit Care Clin 1991; 7:713–723.
45. Drumi W, Polzleitner D, Laggner AN, et al. Dextran-40, acute renal failure, and elevated plasma oncotic pressure. N Engl J Med 1988; 318:252–254.
Colloid-Crystalloid Conundrum
46. Moore FD. The effects of hemorrhage on body composition. N Engl J Med 1965; 273:567–577.
47. Shires T, Carrico J, Lightfoot S. Fluid therapy in hemorrhagic shock. Arch Surg 1964; 88:688–693.
48. Roberts I, Blackhall K, Alderson P, et al. Human albumin solution for resuscitation and volume expansion in critically ill patients. Cochrane Database Syst Rev 2011; 10:CD001208.
49. Perel P, Roberts I. Colloids versus crystalloids for fluid resuscitation in critically ill patients. Cochrane Database Syst Rev 2012; 6:CD000567.
50. Boyd JH, Forbes J, Nakada T-a, et al. Fluid resuscitation in septic shock: A positive fluid balance and elevated central venous pressure are associated with increased mortality. Crit Care Med 2011; 39:259–265.
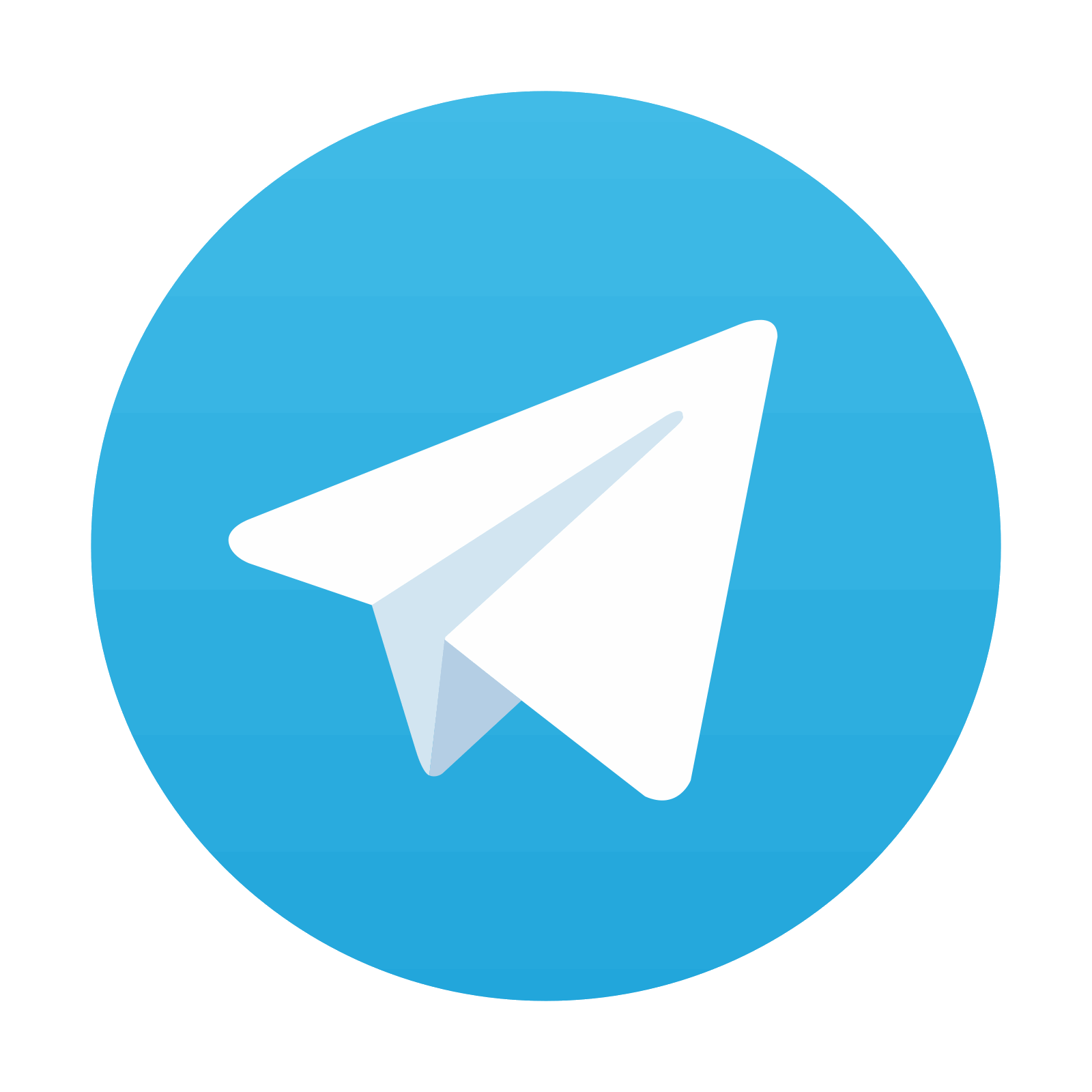
Stay updated, free articles. Join our Telegram channel
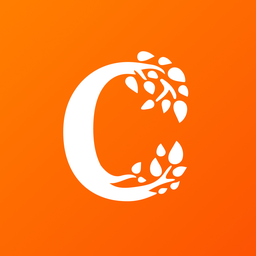
Full access? Get Clinical Tree
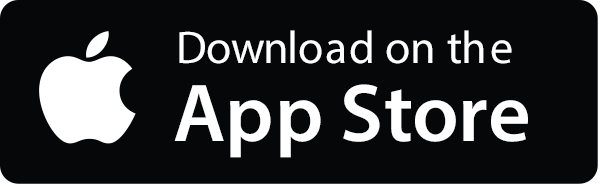
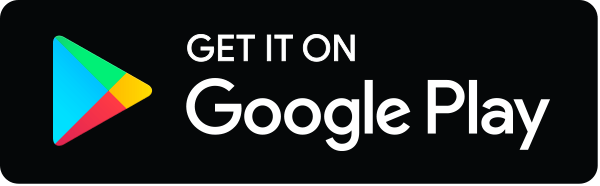