Chapter 41 Ventilation/Perfusion Inequality
The primary function of the lung is to exchange oxygen and carbon dioxide between inspired air and blood. Efficient gas exchange requires the close matching of regional ventilation and perfusion (VA/Q). However, as the largest organ in the body, the lung is influenced by external and internal factors that affect ventilation/perfusion relationships. In children, VA/Q mismatch and intrapulmonary shunt cause most gas exchange abnormalities (Table 41-1). Lung units that are poorly ventilated in relation to blood flow (low VA/Q) produce blood with low oxygen content (desaturated blood), but units with high VA/Q ratios cannot compensate, because the oxygen content of blood leaving these areas has nearly the same oxygen content as that from normal VA/Q regions as a result of the sigmoid shape of the hemoglobin oxygen dissociation curve (Figure 41-1). Early studies supported the concept that vertical gradients of both ventilation and perfusion produced VA/Q matching. Newer studies that achieved greater spatial resolution demonstrated that perfusion and ventilation are rather heterogeneous and that this heterogeneity serves to match ventilation and perfusion.
Table 41–1 Causes of Hypoxemia
Intrapulmonary Factors | Extrapulmonary Factors |
---|---|
PRIMARY | PRIMARY |
Ventilation/perfusion mismatch | Decreased minute ventilationDecreased cardiac output |
Shunt | Decreased Fio2 |
Alveolar-end capillary diffusion limitation | SECONDARY |
Decreased P50 | |
Decreased hemoglobin concentration | |
Alkalosis |
where O2 is O2 consumption, FIO2 is the fraction of inspired O2, A represents alveolar ventilation, and FAO2 is the fraction of alveolar O2. This equation can be rearranged to read:
where PIO2 is the inspired PO2 and PB is barometric pressure. The concept underlying this equation is that alveolar oxygen level (PAO2) is the difference between what comes in (PIO2) and the amount taken up by the pulmonary capillaries [(O2/A)(PB – 47 mm Hg)]. The ratio O2/A can be estimated from a surrogate for the ratio between O2 and A. By using PCO2 in arterial blood as an estimate of alveolar CO2, CO2/A as an estimator of FACO2, and the respiratory quotient R = CO2/O2, the second term of Equation 3 can be estimated by PACO2/R. The alveolar gas equation can then be derived as follows:
Distribution of Ventilation
Ventilation of the lung is heterogeneous and is influenced by multiple factors, including gravity, posture, and even experimental technique. Gravity has been considered predominant because of the variation in pleural pressure from the apex to the base of the lung. The lung is a viscoelastic structure encased in the supporting chest wall, with gravity imposing a globular shape on the lung. Pleural pressure is more negative at the apex of the lung compared with the base, increasing approximately 0.25 cm H2O per centimeter of vertical distance toward the lung base. Thus transpulmonary pressure is more marked at the apex, so apical alveoli are large and at the upper end of the normal pressure volume curve. They distend less for a given pressure change, that is, they are less compliant. In the spontaneously breathing upright human, maximal gas distribution occurs at the base and progressively diminishes toward the lung apex.1,2 This gradient also exists when inhalation occurs in the supine or lateral decubitus position, although to a lesser degree. Fast inspirations from functional residual capacity (at supranormal flow rates >1.5 L/s) may reverse this distribution with preferential ventilation of the upper parts of the lung.3
Distribution of Perfusion
The predominant characteristic of the pulmonary circulation is that it is a low-pressure system. The mean pulmonary artery pressure (PPA) is approximately 15 mm Hg, whereas the mean systemic arterial pressure is on the order of 100 mm Hg. This finding implies that external pressures play a greater role in determining blood flow within the lung. According to the classical model of lung perfusion, gravity affects pulmonary blood flow (PBF) in a similar fashion and to a much greater extent. In general, the dependent areas of lung receive more blood flow. The pulmonary artery pressure decreases by 1 cm H2O per centimeter of vertical distance up the lung, so the driving pressure rapidly approaches zero with minimal blood flow to the apices, and indeed PPA may become negative. In the erect human, blood flow progressively increases from apex to base.4
The three-zone model of PBF has been widely used to explain the heterogeneity of perfusion within the lung (Figure 41-2).4 Three variables comprise the components of this model: pulmonary arterial (PPA), alveolar (PA), and pulmonary venous (PV) pressures. The degree of blood flow within the lung depends on the relative magnitudes of these pressures within that zone. Zone 1 (PA > PPA > Pv) has negligible blood flow, as the higher alveolar pressure is believed to compress collapsible capillaries. This region is one of minimal gas exchange and “wasted” ventilation. Zone 1 conditions are rare except in cases of diminished pulmonary blood flow (e.g., hypotension or cardiac failure) or increased PA encountered during positive pressure ventilation. Zone 2 consists of the mid portions of the lungs in which PPA> PA > PV, where flow rate is determined by the difference between pulmonary arterial and alveolar pressure. Venous pressure does not influence the flow rate. Blood flow progressively increases with descent through this zone as Pa increases, whereas PPA remains relatively constant.
In the lowest zone of the lung described by West et al.,4 zone 3, PPA > PV > PA, therefore the arteriovenous pressure gradient (PPA – PV) determines flow rate. This gradient remains relatively constant as it descends through this zone, although because pleural pressures increase less, blood flow is greater in more dependent areas of zone 3. A zone 4 region in the most dependent areas of lung also has been described. In this region, transudated pulmonary interstitial fluid increases interstitial pressures, thereby reducing blood flow; this effect is exaggerated as lung volume diminishes from total lung capacity to residual volume.
PBF in immature animals differs in several important ways from that in adult animals. Studies in piglets suggest that the pulmonary vascular bed may be fully recruited,5,6 with no contribution of Starling resistors in the pulmonary circulation during exposure to acute or chronic hypoxia. Furthermore, neonatal piglets show a relative hypoxemia and an increased dispersion of PBF (by the multiple inert gas elimination technique [MIGET]). This dispersion is measured as the standard deviation of PBF and is the second moment on a log scale of distribution about the blood flow mean among both high and low VA/Q units compared with mature animals. In general, an increase in the standard deviation of PBF also occurs with lung disease.
Fractal Model of Pulmonary Blood Flow and Ventilation
The gravitational model provided a useful and accurate model of PBF. However, as studies employed increasing power of resolution, PBF was shown to have greater heterogeneity than was described by the gravitational model.7–9 Blood flow in isogravitational planes was shown to be nearly as heterogeneous as the entire lung, yet the gravitational model predicted uniform flow in such planes (Figure 41-3). This heterogeneity in PBF was initially considered random, but the regional flow distributions were shown to be correlated with neighboring regions.9 Thus high-flow regions were adjacent to other high-flow regions and low-flow regions bordered other low-flow regions. The distribution of PBF was shown to be independent of the scale of measurement, suggesting a fractal nature of PBF.
< div class='tao-gold-member'>
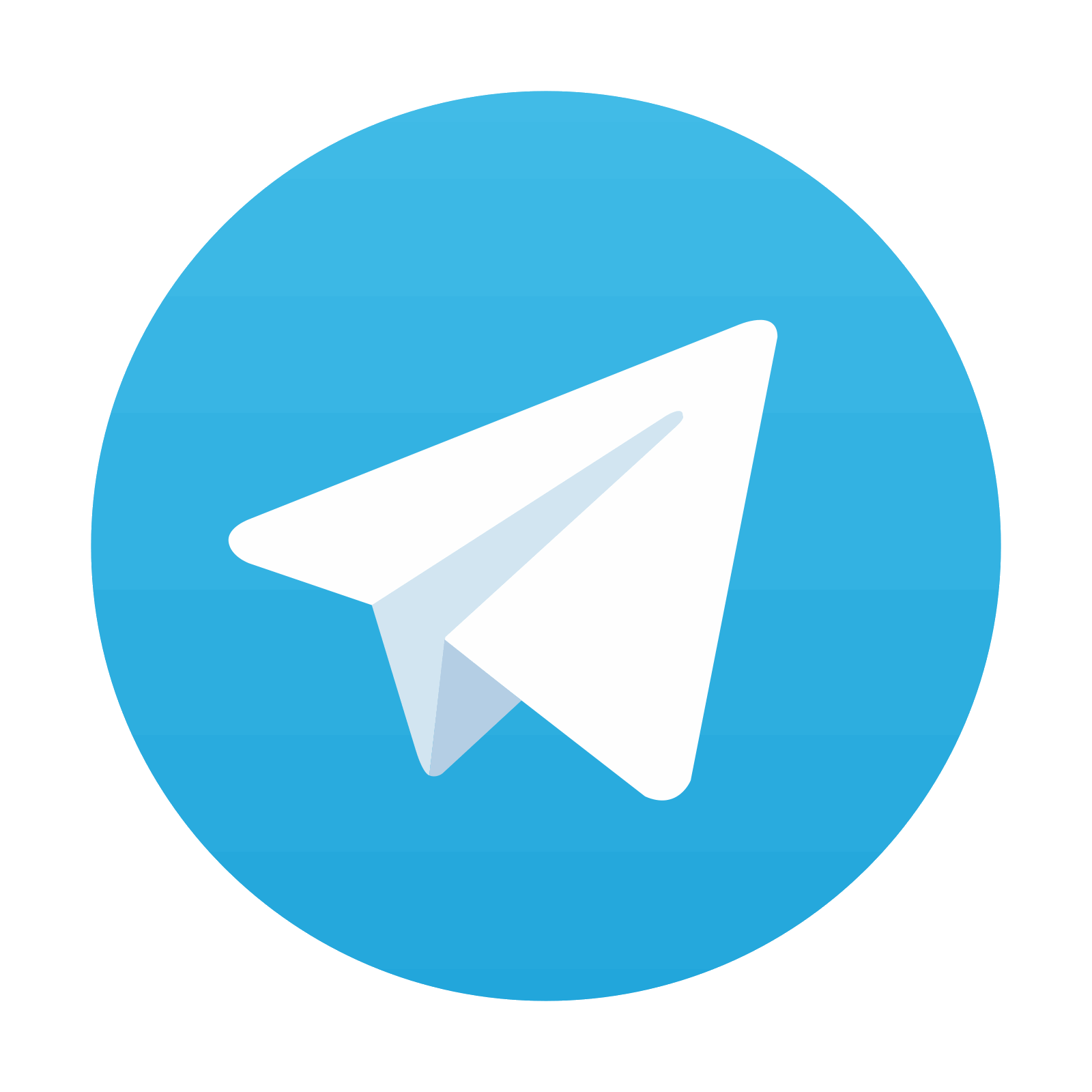
Stay updated, free articles. Join our Telegram channel
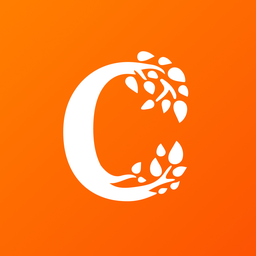
Full access? Get Clinical Tree
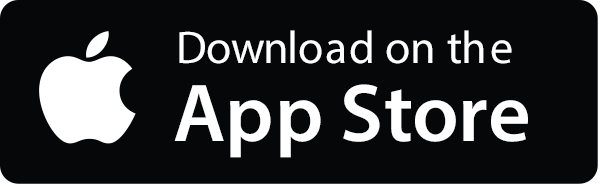
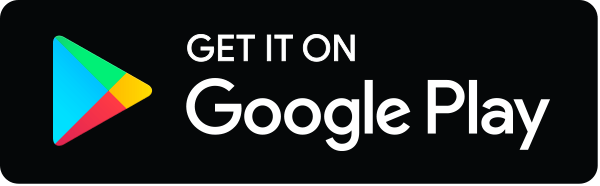