Chapter 31 Critical Care After Surgery for Congenital Cardiac Disease
Congenital anomalies account for the largest diagnostic category among causes of infant mortality in the United States.1 Structural heart disease leads the list of congenital malformations. Of the more than 4 million children born each year in the United States, nearly 40,000 have some form of congenital heart disease (CHD). Approximately half of these children appear for therapeutic intervention within the first year of life, and the vast majority of them require critical care expertise. Patients with congenital or acquired heart disease compose a major diagnostic category for admissions in large pediatric intensive care units (ICUs) across the country, representing 30% to 40% or more of ICU admissions in many centers.
Newborn Considerations
Care of the critically ill neonate requires an appreciation of the special structural and functional features of immature organs, the interactions of the “transitional” neonatal circulation, and the secondary effects of the congenital heart lesion on other organ systems.2–4 The neonate appears to respond more quickly and profoundly to physiologically stressful circumstances, which may be expressed in terms of rapid changes in pH, lactic acid, blood glucose, and temperature. Neonates have diminished fat and carbohydrate reserves compared with older children. The higher metabolic rate and oxygen consumption of the neonate play a significant role in the rapid onset of hypoxemia when these patients become apneic. Immaturity of the liver and kidney may be associated with reduced protein synthesis and glomerular filtration such that drug metabolism is altered and hepatic synthetic function is reduced. These issues may be compounded by the normal increased total body water of the neonate compared with the older patient, along with the propensity of the capillary system of the neonate to leak fluid from the intravascular space. This is especially prominent in the lung of the neonate in whom the pulmonary vascular bed is nearly fully recruited at rest, and lymphatic recruitment required to handle increased mean capillary pressures associated with increases in pulmonary blood flow may be unavailable.4 The neonatal myocardium is less compliant than that of the older child, is less tolerant of increases in afterload, and is less responsive to increases in preload. Younger age also predisposes the myocardium to the adverse effects of cardiopulmonary bypass (CPB) and hypothermic ischemia implicit in support techniques used during cardiac surgery. These factors do not preclude intervention in the neonate but simply dictate that extraordinary vigilance be applied to the care of these children and that intensive care management plans take into account their immature physiology.
The observed benefits of neonatal reparative operations in patients with two ventricles are numerous (Box 31-1). They continue to dictate that care of the newborn with complex CHD after CPB be a central feature of cardiac intensive care. Elimination of cyanosis and congestive heart failure (CHF) early in life optimize, conditions for normal growth and development. Palliative procedures such as pulmonary artery bands and systemic-to-pulmonary artery shunts may not fully address cyanosis or CHF and may introduce their own set of physiologic and anatomic complications. Some examples of improved outcomes with a single reparative operation rather than staged palliation as a newborn are well known, are supported by published literature, and evoke little controversy. Approaches that have been abandoned include banding the pulmonary arteries in truncus arteriosus,5 staging repair of type B interrupted aortic arch (IAA),6 and staging rather than repairing in a single session transposition of the great arteries with IAA.7 In other conditions (e.g., severely cyanotic newborn with tetralogy of Fallot [TOF]), the risks and benefits of neonatal repair versus a palliative shunt are debatable.8
Whereas the neonate may be more labile than the older child, there is ample evidence that this age group is more resilient in its response to metabolic or ischemic injury. In fact, the neonate may be particularly capable of coping with some forms of stress. Tolerance of hypoxemia in the neonate is characteristic of many species,9 and the plasticity of the neurologic system in the neonate is well known. Neonates with obstructive left heart lesions often present with profound metabolic acidosis but can be effectively resuscitated without persistent organ system impairment or sequelae as the rule rather than the exception. The pliability and mobility of vascular structures in the neonate improve the technical aspects of surgery. Reparative operations in neonates take advantage of normal postnatal changes, allowing more normal growth and development in crucial areas such as myocardial muscle, pulmonary parenchyma, and coronary and pulmonary angiogenesis. Postoperative pulmonary hypertensive events are more common in the infant who has been exposed to weeks or months of high pulmonary pressure and flow.5,10 This seems especially true for such lesions as truncus arteriosus, complete atrioventricular (AV) canal defects, and transposition of the great arteries with ventricular septal defects (VSDs). Finally, cognitive and psychomotor abnormalities associated with months of hypoxemia or abnormal hemodynamics may be diminished or eliminated by early repair. However, if early reparative surgery results in more exposures to CPB (e.g., repeated conduit changes) and any associated cognitive or subtle adverse effects on motor function, then the risk-to-benefit assessment needs to be modified accordingly.
Preoperative Care
Physical Examination and Laboratory Data
A complete history and physical examination are required, with special attention directed to the extent of cardiopulmonary impairment, airway abnormalities, and associated extracardiac congenital anomalies.11 Intrathoracic and extrathoracic airway problems in patients with Down syndrome, disorders of calcium homeostasis and immunologic deficiencies in patients with aortic arch abnormalities, and renal abnormalities in patients with esophageal atresia and CHD are a few of the associated congenital abnormalities with which the anesthesiologist should be familiar. Intercurrent pulmonary infection is a common and significant finding in chronically overcirculated lungs. The presence, degree, and duration of hypoxemia are important details that, in the absence of iron deficiency, are reflected in the hematocrit. The nadir of physiologic anemia during infancy may contribute to left-to-right shunting by decreasing the relative pulmonary vascular resistance (PVR).12
Chest radiography can be used to asses heart size, pulmonary vascular congestion, airway compression, and areas of consolidation or atelectasis. The electrocardiogram (ECG) may reveal rhythm disturbances and demonstrate ventricular strain patterns (ST and T-wave changes) characteristic of unphysiologic pressure or volume burdens on the ventricles. Electrolyte abnormalities caused by CHF and forced diuresis also must be evaluated preoperatively. Severe hypochloremic metabolic alkalosis may occur in some patients. It is important to discontinue digoxin preoperatively and to avoid hyperventilation and administration of calcium to these patients during induction of anesthesia, because the alkalotic hypokalemic, hypercalcemic, hypotensive, dilated, digoxin-bound myocardium fibrillates with ease.
Echocardiographic and Doppler Assessment
Advances in echocardiographic imaging have had an enormous impact on the diagnosis of CHD.13 Accurate anatomic diagnosis now is routine in children without the need for cardiac catheterization. Echocardiography is the preferred imaging modality for assessment of intracardiac anatomic features in young children. However, one should be aware of the current limitations of echocardiographic and Doppler techniques so that alternative diagnoses can be considered when intraoperative or postoperative findings are inconsistent with the working echocardiographic diagnosis.
Cardiac Catheterization
Normal intracardiac pressure and saturation values in children are described in Chapter 24. Normally, there is no significant change in oxygen saturation from vena cava to pulmonary artery. In the child with CHD, the superior vena cava (SVC) gives the best indication of true mixed venous oxygen saturation; a 5% or greater step-up in saturation downstream suggests the presence of a left to right shunt.14 It would occur at the level of the right atrium with an ASD, in the right ventricle with a VSD, and in the pulmonary artery with a patent ductus arteriosus (PDA). The magnitude of the left-to-right shunt can be calculated from the Fick equation. The oxygen consumption of the patient usually is measured, as are the saturation values, but subsequent flow and resistance calculations can be in error. The frequently used term Qp/Qs (pulmonary-to-systemic blood flow ratio) can be derived simply from the measured oxygen saturation values.
In the presence of a left-to-right shunt and elevated PVR, pressure and saturation measurements often are repeated, with the patient breathing 100% oxygen to assess both the reactivity of the pulmonary vascular bed and any contribution of ventilation-perfusion abnormalities to hypoxemia. If breathing 100% oxygen increases pulmonary blood flow and dramatically increases Qp/Qs (with a fall in PVR), potentially reversible processes such as hypoxic pulmonary vasoconstriction probably are contributing to the elevated PVR. The patient with a high, unresponsive PVR and a small left-to-right shunt despite a large shunt orifice may have extensive pulmonary vascular damage from irreversible obstructive pulmonary vascular disease. If so, surgical repair usually is contraindicated if the child is older than 1 year.15
During cardiac catheterization, anatomic abnormalities are identified angiographically. Special angled views provide specific information about the location and extent of congenital defects.16 Ventricular function is assessed angiographically and physiologically (e.g., by pressure measurements). The calculated size of a cardiac chamber may have an important bearing on its ability to support the circulation of a child with hypoplastic ventricles.
Magnetic Resonance Imaging and Angiography
Magnetic resonance imaging (MRI) and magnetic resonance angiography (MRA) have emerged as important diagnostic modalities in the evaluation of the cardiovascular system after the development of ECG-gated MRI. Image acquisition is triggered to the patient’s ECG to counter motion artifacts and to acquire cine sequences that allow imaging of cardiac structures and visualization of blood flow throughout the cardiac cycle. In addition to providing excellent anatomical and three-dimensional images, particularly of the pulmonary veins and thoracic aorta, it also is possible with MRA to qualitatively assess valve and ventricular function and to quantify flow, ventricular volume, mass, and ejection fraction.17,18 Whereas ferromagnetic implants near the region of interest might produce artifact, sternal wires and vascular clips produce relatively minor disturbances; therefore MRI can be performed in patients who have undergone previous cardiac surgery. Contraindications include patients with pacemakers, recently implanted endovascular or intracardiac implants, and aneurysm clips on vessels that will be exposed directly to the magnetic field.
Assessment of Patient Status and Predominant Pathophysiology
Severe Hypoxemia
Many of the cyanotic forms of CHD present in the ICU with severe hypoxemia (PaO2 <50 mm Hg) during the first few days of life, but without respiratory distress. Infusion of prostaglandin E1 (PGE1) in patients with decreased pulmonary blood flow maintains or reestablishes pulmonary flow through the ductus arteriosus. This may also improve mixing of venous and arterial blood at the atrial level in patients with transposition of the great arteries.19 Consequently, neonates rarely require surgery while they are severely hypoxemic. During preoperative preparation with PGE1, neurologic examination and blood chemistry analysis of renal, hepatic, and hematologic function are necessary to assess the effects of severe hypoxemia during or after birth on end-organ dysfunction.
PGE1 dilates the ductus arteriosus of the neonate with life-threatening ductus-dependent cardiac lesions and improves the patient’s condition before surgery. PGE1 can reopen a functionally closed ductus arteriosus for several days after birth, or it can maintain patency of the ductus arteriosus for several months postnatally.19,20 The common side effects of PGE1 infusion—apnea, hypotension, fever, CNS excitation—are easily managed in the neonate when normal therapeutic doses of the drug (0.02–0.05 μg/kg/min) are used.21 However, PGE1 is a potent vasodilator, so intravascular volume frequently requires augmentation. Patients with intermittent apnea resulting from administration of PGE1 may require mechanical ventilation preoperatively.
PGE1 usually improves the arterial oxygenation of hypoxemic neonates who have poor pulmonary perfusion as a result of obstructed pulmonary flow (critical pulmonic stenosis or pulmonary atresia). By providing pulmonary blood flow from the aorta via the ductus arteriosus, an infusion of PGE1 improves oxygenation and stabilizes the condition of neonates with these lesions. The improved oxygenation reverses the lactic acidosis that may have developed during episodes of severe hypoxia. PGE1 administration for 24 hours usually markedly improves the condition of a severely hypoxemic neonate with restricted pulmonary blood flow.22
Excessive Pulmonary Blood Flow
Excessive pulmonary blood flow is frequently the primary problem of patients with CHD. The intensivist must carefully evaluate the hemodynamic and respiratory impact of left-to-right shunts and the extent to which it contributes to the perioperative course in the ICU. Children with left-to-right shunts may have chronic low-grade pulmonary infection and congestion that cannot be eliminated despite optimal preoperative preparation. If so, surgery should not be postponed further. Respiratory syncytial viral infections are particularly prevalent in this population, but improvements in intensive care have markedly improved outcome with this and other viral pneumonias.23
Children with failing hearts increase endogenous catecholamine production and redistribute cardiac output to favored organs by their increased heart rate and decreased extremity perfusion. In the most severe cases, the evaluation reveals a child whose body weight is below the third percentile for age and who is tachypneic, tachycardic, and dusky in room air. The child may have intercostal and substernal retractions and skin that is cool to the touch. Capillary refill may be prolonged. Expiratory wheezes usually are audible. Medical management with digoxin and diuretics may improve the patient’s condition, but the diuretics may induce profound hypochloremic alkalosis and potassium depletion that often persist after surgery.
Obstruction of Left Heart Outflow
Ductal closure in the neonate with these problems causes dramatic worsening of the patient’s condition. The patient becomes critically ill or even moribund and requires PGE1 infusion (see previous section) for survival. PGE1 allows blood flow into the aorta from the pulmonary artery because it maintains the patency of the ductus arteriosus.22,24 PGE1 infusion improves perfusion and metabolism in neonates with acidosis, metabolic derangements, and renal failure because of inadequate systemic perfusion, so surgery generally can be deferred until the patient’s condition improves. Ventilatory and inotropic support and correction of metabolic acidosis, along with calcium, glucose, and electrolyte abnormalities are often indicated preoperatively. The stabilization period also allows assessment of the magnitude of end-organ dysfunction caused by the preceding period of inadequate systemic perfusion. Adequacy of resuscitation, rather than severity of illness at presentation, appears to influence postoperative outcome.25
Postoperative Care
Assessment
When the clinical course of patients after cardiac surgery deviates from the usual expectation of uncomplicated recovery, our first responsibility is to verify the accuracy of the preoperative diagnosis and the adequacy of surgical repair. For example, a young infant who is acidotic, hypotensive, and cyanotic after surgical repair of TOF may tempt us to ascribe these findings to the vagaries of ischemia/reperfusion injury of CPB or transient, postoperative stiffness of the right ventricle. However, the real culprit may be an additional VSD undetected preoperatively and therefore not closed, a residual VSD around the surgical patch, or residual RV outflow obstruction. Any of these anatomic issues and more can produce serious adverse outcomes. Getting the right postoperative assessment is imperative and treatment follows accordingly. Evaluation of the postoperative patient relies on examination, monitoring, interpretation of vital signs, or other bedside data and imaging (Box 31-2). When the accuracy of the diagnosis and adequacy of the repair are established, then a low cardiac output state can be presumed and treatment optimized. Treating low cardiac output states and preventing cardiovascular collapse often are the central features of pediatric cardiac intensive care and are the focus of this chapter.
Box 31–2 Ten Intensive Care Strategies to Diagnose and Support Low Cardiac Output States
The initial assessment after cardiac surgery begins with review of the operative findings. This includes details of the operative repair and CPB, particularly total CPB or myocardial ischemia (aortic cross-clamp) times; concerns about myocardial protection; recovery of myocardial contractility; typical postoperative systemic arterial and central venous pressures; findings from intraoperative transesophageal echocardiography, if performed; and vasoactive medication requirements. This information guides subsequent examination, which should focus on the quality of the repair or palliation plus clinical assessment of cardiac output (Box 31-3). In addition to a complete cardiovascular examination, a routine set of laboratory tests should be obtained, including a chest radiograph, 12- or 15-lead ECG, blood gas analysis, serum electrolytes and glucose, ionized calcium and lactate measurements, complete blood count, and coagulation profile.
Monitoring
Possible causes of abnormally elevated LA pressure are listed in Box 31-4. In addition to pressure data, intracardiac catheters in the RA (or a percutaneously placed central venous catheter), LA, and PA can be used to monitor the oxygen saturation of systemic venous or pulmonary venous blood.
Box 31–4 Common Causes of Elevated Left Atrial Pressure After Cardiopulmonary Bypass
Table 31-1 lists the causes of abnormally high or low RA, LA, and PA oxygen saturations, which can be measured at the bedside in the ICU. After reparative surgery, patients with no intracardiac shunts and adequate cardiac output may have a mild reduction in RA oxygen saturation to approximately 60%. Lower RA oxygen saturation does not necessarily indicate low cardiac output, if a patient has arterial desaturation (common mixing lessons, lung diseases, etc.) and the arteriovenous oxygen difference is normal at 25%, there may be appropriate oxygen delivery and extraction. Elevated RA oxygen saturation often is the result of left-to-right shunting at the atrial level (e.g., from the left atrium, anomalous pulmonary vein, or left ventricular [LV]-to-RA shunt). Blood in the left atrium normally is fully saturated with oxygen (i.e., approximately 100%). The two chief causes of reduced LA oxygen saturation are an atrial level right-to-left shunt and pulmonary venous desaturation from abnormal gas exchange.
Table 31–1 Causes of Abnormal Right Atrial, Left Atrial, or Pulmonary Artery Oxygen Saturation
Location | Elevated | Reduced |
---|---|---|
RA | Atrial level left-to-right shunt | ↑Vo2 (e.g., low CO, fever) |
Anomalous pulmonary venous return | ↓Sao2 saturation with a normal A-V O2 difference | |
Left ventricular-to-right atrial shunt | Anemia | |
↑Dissolved O2 content | Catheter tip position (e.g., near CS) | |
↓O2 extraction | ||
Catheter tip position (e.g., near renal veins) | ||
LA | Does not occur | Atrial level right-to-left shunt |
↓Pvo2 (e.g., parenchymal lung disease) | ||
PA | Significant left-to-right shunt | ↑O2 extraction (e.g., low CO, fever) |
Small left-to-right shunt with incomplete mixing of blood | Sao2 saturation with a normal A-V O2 difference | |
Catheter tip position (e.g., PA “wedge”) | Anemia |
A-V, Arteriovenous; CO, cardiac output; CS, coronary sinus; LA, left atrium; PA, pulmonary artery; Pvo2, pulmonary vein oxygen tension; RA, right atrium; Sao2, arterial oxygen saturation; Vo2, oxygen consumption.
In the absence of left-to-right shunts, PA oxygen saturation is the best representation of the “true” mixed venous oxygen saturation because all sources of systemic venous blood should be thoroughly combined as they are ejected from the right ventricle. When elevated, this saturation is useful in identifying residual left-to-right shunts after repair of VSD(s). The absolute value of the PA oxygen saturation is a predictor of significant postoperative residual shunt. In patients after TOF or VSD repair, PA oxygen saturation greater than 80% within 48 hours of surgery with supplemental O2 at a fractional inspired oxygen concentration (Fio2) less than 0.5 is a sensitive indicator of significant left-to-right shunt (Qp/Qs >1.5) 1 year after surgery.26 Determination of PA oxygen saturation also can be useful in patients with systemic-to-pulmonary artery collaterals because flow from these vessels into the pulmonary arteries can increase oxygen saturation.
Low Cardiac Output Syndrome
Although some causes of low cardiac output after CPB are attributable to residual or undiagnosed structural lesions, progressive low cardiac output states do occur. Several factors have been implicated in the development of myocardial dysfunction after CPB including (1) the inflammatory response associated with CPB, (2) the effects of myocardial ischemia from aortic cross-clamping, (3) hypothermia, (4) reperfusion injury, (5) inadequate myocardial protection, and (6) ventriculotomy (when performed). The expression and prevention of reperfusion injury after aortic cross-clamping on CPB is the subject of intense investigation. The typical decrease in cardiac index in newborns after an arterial switch operation (ASO) has been well characterized (Figure 31-1).27 In a group of 122 newborns, the median maximal decrease in cardiac index that typically occurred 6 to 12 hours after separation from CPB was 32%. One fourth of these newborns reached a nadir of cardiac index that was less than 2 L/min/m2 on the first postoperative night. Low cardiac output syndrome (LCOS) does occur in the postoperative patient, but appropriate anticipation and intervention can do much to avert morbidity or the need for mechanical support. Signs of low cardiac output are listed in Box 31-3. Mixed venous oxygen saturation, whole blood pH, and lactate are laboratory measures commonly used to evaluate the adequacy of tissue perfusion and hence cardiac output.
Right Ventriculotomy and Restrictive Physiology
RV “restrictive” physiology in infants and children who have undergone congenital cardiac surgery has been demonstrated by echocardiography as persistent antegrade diastolic blood flow into the pulmonary circulation after reconstruction of the RV outflow. This occurs in the setting of elevated RV end-diastolic pressure and RV hypertrophy, and the right ventricle demonstrates diastolic dysfunction with an inability to relax and fill during diastole. The right ventricle usually is not dilated in this circumstance, and pulmonary regurgitation is limited because of the higher diastolic pressure in the right ventricle.29
This concept has been extended to older patients with single-ventricle physiology who are at high risk for Fontan operations.30 The Fontan circulation relies on passive flow of blood through the pulmonary circulation without benefit of a pulmonary ventricle. If an atrial septal communication or fenestration is left at the time of the Fontan procedure, the resulting right-to-left shunt helps to preserve cardiac output. These children have fewer postoperative complications.31 It is better to shunt blood right to left and accept some decrement in oxygen saturation but maintain ventricular filling and cardiac output rather than have high oxygen saturation but low blood pressure and cardiac output.
Pharmacologic Support
Catecholamines
Preload adjustments often do not provide adequate cardiac output. Use of pharmacologic agents to support cardiac output is common.32,33 Table 31-2 lists common vasoactive drugs used in the ICU and their actions. Many prefer to use dopamine first in doses of 3 to 10 μg/kg/min. Dosages greater than 15 μg/kg/min are rarely used because of the known vasoconstrictor and chronotropic properties of dopamine at very high doses. However, extreme biologic variability in pharmacokinetics and pharmacodynamics defies placing narrow limits on recommended dosages. Dobutamine’s chronotropic and vasodilatory advantages recognized in adults with coronary artery disease have not always proved equally efficacious in clinical studies in children. The significant chronotropic effect and increased oxygen consumption induced by isoproterenol have also increasingly limited its use in neonates and infants. Epinephrine is occasionally useful for short-term therapy when high systemic pressures are sought, provided the temporary increase in peripheral vascular resistance can be tolerated. High doses of epinephrine occasionally are necessary to increase pulmonary blood flow across significantly narrowed systemic-to-pulmonary artery shunts when oxygen saturations are low and falling. Arginine vasopressin has been advocated for states of refractory vasodilation associated with low circulating vasopressin levels as may rarely occur after CPB in children.34 Vasopressin has been used to treat low systemic blood pressure in postoperative pediatric cardiac patients with pulmonary hypertension where it may ameliorate hypoxic pulmonary vasoconstriction and not exacerbate pulmonary hypertension.35
In the past, the side effects of inotropic support of the heart with catecholamines seemed a lesser concern in children than in adults with an ischemic, noncompliant heart. Tachycardia, an increased end-diastolic pressure and afterload, and increased myocardial oxygen consumption, despite their undesirable side effects, were tolerated by most children in need of inotropic support after CPB. However, with increasing perioperative experience in neonates and young infants, the adverse effects of vasoactive drugs have become more evident. The less compliant neonatal myocardium, such as the ischemic adult heart, may raise its end-diastolic pressure during higher doses of dopamine infusion or may develop even more extreme noncompliance. Actual myocardial necrosis caused by high doses of epinephrine infusions has been identified in neonatal animal models after CPB.36,37 Although these agents do increase the cardiac output, the concomitant increase in ventricular filling pressure is less well tolerated by the immature myocardium than it is in older children. Many of the complex corrective procedures performed in neonates and small infants are accompanied by transient postoperative arrhythmias that are either induced or exacerbated by catecholamines, which can have a profound adverse effect on the patient’s recovery after surgery. Diastolic function is crucial in older patients with single ventricles and can be adversely affected by catecholamines. Nevertheless, the predictable and often significant decrease in cardiac output documented by many investigators after CPB in infants and older children continues to justify the practice of judiciously using catecholamines to support the heart and circulation while weaning them from CPB and during the immediate postoperative period.
Type III Phosphodiesterase Inhibitors
Milrinone has emerged as an important inotropic agent for use in children after open heart surgery.38–40 It is a nonglycosidic, noncatecholamine inotropic agent with additional vasodilatory and lusitropic properties used extensively in adults for treatment of heart failure and now also abundantly used in pediatric practice. This class of drugs exerts its principal effects by inhibiting phosphodiesterase III, the enzyme that metabolizes cyclic adenosine monophosphate (cAMP). By increasing intracellular cAMP, calcium transport into the cell is favored, and the increased intracellular calcium stores enhance the contractile state of the myocyte. In addition, reuptake of calcium is a cAMP-dependent process, and these agents may enhance diastolic relaxation of the myocardium (lusitropy) by increasing the rate of calcium reuptake after systole. The drug also appears to work synergistically with low doses of β-agonists and has fewer side effects than other catecholamine vasodilators, such as isoproterenol. In critically ill postoperative newborns, milrinone increases cardiac output, lowers filling pressures, and reduces pulmonary artery pressures.39
The Prophylactic Intravenous Use of Milrinone after Cardiac Operation in Pediatrics (PRIMACORP) trial investigated the efficacy and safety of prophylactic milrinone use to prevent LCOS after cardiac surgery in high-risk pediatric patients.40 The study was a multicenter, randomized, double-blind, placebo-controlled trial using three parallel treatment groups (low-dose: 25 μg/kg bolus over 60 minutes followed by a 0.25 μg/kg/min infusion for 35 hours; high-dose: 75 μg/kg bolus followed by 0.75 μg/kg/min; or placebo). The composite endpoint of death or the development of LCOS was evaluated at 36 hours and at the follow-up visit. Among 238 treated patients, the prophylactic use of high-dose milrinone significantly reduced the risk of death or the development of LCOS relative to placebo with a relative risk reduction of 55% (P = .023) in the treated patients (Figure 31-2). Patients who developed LCOS had a significantly longer cumulative duration of mechanical ventilation and hospital stay in comparison with those who did not develop LCOS. The authors concluded that the prophylactic use of high-dose milrinone after pediatric congenital heart surgery reduces the risk of LCOS. Dopamine and milrinone have emerged as our most commonly used inotropic agents, often used in combination to achieve increased cardiac output, maintain arterial perfusion pressure, and improve diastolic relaxation.
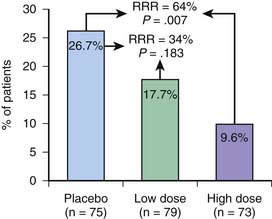
Figure 31–2 Primary end point: development of low cardiac output syndrome (LCOS) or death in the first 36 hours.
(From Hoffman TM, Wernovsky G, Atz AM, et al: Efficacy and safety of milrinone in preventing low cardiac output syndrome in infants and children after corrective surgery for congenital heart disease, Circulation 107:996-1002, 2003.)
Thyroid Hormone
LCOS typically overlaps with the time that free and total triiodothyronine (T3) levels are significantly suppressed after surgical reconstruction, namely, during the first 24 to 48 hours postoperatively. This is a significant observation, as T3 is the predominant form of biologically active thyroid hormone and is known to improve cardiac output by improving the inotropic state of animal and human hearts while decreasing systemic vascular resistance. Limited studies of T3 supplementation after cardiac surgery have been performed in children. Mainwaring et al.41 gave two bolus doses of T3 after the Fontan procedure to 10 children ages 19 to 42 months. Compared with a historical control group, the T3 patients had a significantly shorter period of mechanical ventilation. Bettendorf et al.42 randomized 40 children undergoing a wide variety of cardiac procedures to receive bolus dosing of T3 or placebo. Cardiac output reportedly was higher in the treatment group but was estimated by echocardiography. Chowdhury et al.43 randomized 28 children aged 0 to 18 years to a 5-day continuous infusion of T3 (0.05-0.15 μg/kg/hour) or placebo. Among neonates, the T3 group had lower severity of illness scores and lower inotrope requirements. The T3 group also had a trend toward higher mixed venous oxygen saturations, fewer days of mechanical ventilation, and a shorter postoperative length of stay. No adverse effects of T3 administration were recorded in any of these small series.
Thus the current literature demonstrates that infants undergoing cardiac surgery experience significant depression of T3 levels and that supplementation of T3 may have an impact on physiologic variables if not other measures of improved outcomes. Larger trials with more rigorous trial designs are under way and may address whether certain subgroups at particular risk for LCOS may benefit from T3 administration. At this time, the routine use of T3 is not supported by the existing literature.44
Other Afterload-Reducing Agents
The natriuretic hormone system is an important regulator of neurohumoral activation, vascular tone, diastolic function, and fluid balance. Preliminary data suggest that the endogenous biologic activity of the natriuretic hormone system is decreased after pediatric CPB. In theory, infusions of brain natriuretic peptide could oppose the neurohumoral mechanism associated with vasoconstriction and fluid retention after pediatric CPB. In randomized adult studies after CPB, natriuretic hormone infusions suppress the renin-angiotensin-aldosterone axis and improve cardiac loading conditions, cardiac index, and urine output.45,46 Several services in North America have now accrued experience with nesiritide infusions in children.
Levosimendan is a calcium sensitizer that enhances the contractile state of the ventricle by increasing myocyte sensitivity to calcium and induces vasodilation. Levosimendan increases cardiac output by increasing stroke volume. It is independent of cAMP pathways that characterize the mechanism of action of both the catecholamines and the type III phosphodiesterase inhibitors. With its positive inotropic effects, levosimendan may be of value as adjunctive therapy to other inotropic drugs in patients who are refractory or tachyphylactic to other forms of inotropic support. Its hemodynamic effect in children is uncertain, but its pharmacokinetic profile seems similar to adults.47
Other Strategies
Newer strategies to support low cardiac output associated with cardiac surgery in children include use of atrio-biventricular pacing for patients with complete heart block or prolonged interventricular conduction delays and asynchronous contraction.48 Appreciation of the hemodynamic effects of positive and negative pressure ventilation may assist cardiac output. Avoidance of hyperthermia and even induced hypothermia may provide end-organ protection during periods of low cardiac output. Antiinflammatory agents including monoclonal antibodies, competitive receptor blockers, inhibitors of compliment activation, and preoperative preparation with steroids are being actively investigated in an effort to prevent and protect major organs from ischemic injury imposed by CPB and the reperfusion injury associated with the recovery period.
Diastolic Dysfunction
Occasionally there is an alteration of ventricular relaxation, an active energy-dependent process, which reduces ventricular compliance. This is particularly problematic in patients with a hypertrophied ventricle undergoing surgical repair, such as TOF or Fontan surgery, and after CPB in some neonates when myocardial edema may significantly restrict diastolic function (i.e., “restrictive physiology”).28,29 The ventricular cavity size is small, and the stroke volume is decreased. β-Adrenergic antagonists and calcium channel blockers add little to the treatment of this condition. In fact, hypotension or myocardial depression produced by these agents often outweigh any gain from slowing the heart rate. Calcium channel blockers are relatively contraindicated in neonates and small infants because of their dependence on transsarcolemmal flux of calcium to both initiate and sustain contraction.
A gradual increase in intravascular volume to augment ventricular capacity, in addition to the use of low doses of inotropic agents, has proved to be of modest benefit in patients with diastolic dysfunction. Tachycardia must be avoided to optimize diastolic filling time and decrease myocardial oxygen demands. If low cardiac output continues despite treatment, therapy with vasodilators can be carefully attempted to alter systolic wall tension (afterload) and thus decrease the impediment to ventricular ejection. Because the capacity of the vascular bed increases after vasodilation, simultaneous volume replacement is often indicated. Milrinone or enoximone is useful under these circumstances because these agents are noncatecholamine so-called inodilators with vasodilating and lusitropic (improved diastolic state) properties, in contrast with other inotropic agents. Nesiritide also may play a particularly important role in lowering LV filling pressures in patients with heart failure.
Managing Acute Pulmonary Hypertension in the Intensive Care Unit
Children with many forms of CHD are prone to develop perioperative elevations in PVR.49 This situation may complicate the postoperative course, when transient myocardial dysfunction requires optimal control of RV afterload.50
Several factors peculiar to CPB may raise PVR: Pulmonary vascular endothelial dysfunction, microemboli, pulmonary leukostasis, excess thromboxane production, atelectasis, hypoxic pulmonary vasoconstriction, and adrenergic events all have been suggested to play a role in postoperative pulmonary hypertension. Postoperative pulmonary vascular reactivity has been related not only to the presence of preoperative pulmonary hypertension and left-to-right shunts but also to the duration of total CPB. Treatment of postoperative pulmonary hypertensive crises has been partially addressed by surgery at earlier ages, pharmacologic intervention, and other postoperative management strategies (Table 31-3).
Table 31–3 Critical Care Strategies for Postoperative Treatment of Pulmonary Hypertension
Encourage | Avoid |
---|---|
Anatomic investigation | Residual anatomic disease |
Opportunities for right-to-left shunt as “pop-off” | Intact atrial septum in right heart failure |
Sedation/anesthesia | Agitation/pain |
Moderate hyperventilation | Respiratory acidosis |
Moderate alkalosis | Metabolic acidosis |
Adequate inspired oxygen | Alveolar hypoxia |
Normal lung volumes | Atelectasis or overdistention |
Optimal hematocrit | Excessive hematocrit |
Inotropic support | Low output and coronary perfusion |
Vasodilators | Vasoconstrictors/increased afterload |
Pulmonary Vasodilators
Many IV vasodilators have been used with variable success in patients with pulmonary hypertensive disorders requiring critical care. Older style vasodilators such as tolazoline, phenoxybenzamine, nitroprusside, or isoproterenol had little biologic basis for selectivity or enhanced activity in the pulmonary vascular bed.51 However, if myocardial function is depressed and the afterload reducing effect on the left ventricle is beneficial to myocardial function and cardiac output, then these drugs may be of some value. However, in addition to drug-specific side effects, they all have the limitation of potentially profound systemic hypotension, critically lowering right (and left) coronary perfusion pressure and simultaneously increasing intrapulmonary shunt. Even with selective infusions of rapidly metabolized, intravenously administered vasoactive drugs into the pulmonary circulation, systemic drug concentrations and systemic hemodynamic effects can be appreciable.
Prostacyclin appears to have somewhat more selectivity for the pulmonary circulation but at high doses can precipitate a hypotensive crisis in unstable postoperative patients with refractory pulmonary hypertension. It is best suited for chronic outpatient therapy in severe forms of primary pulmonary hypertension.52–54 Agents that improve ventricular function in addition to reducing afterload (e.g., type III phosphodiesterase inhibitors) are more appealing when cardiac output is low.
As an alternative approach to nonspecific vasodilators, it seems logical to target vasoconstrictors known to be associated with pathologic states or critical events. In this regard, endothelin, a potent vasoconstrictor, is elevated in persistent pulmonary hypertension of the newborn, in children with CHD, and in patients after CPB, and seems a likely candidate for investigation of specific receptor blockers. Petrossian et al.55 showed promising amelioration of postoperative pulmonary hypertension associated with CPB in animal models of increased pulmonary blood flow (from intracardiac shunts) when pretreated with endothelin-A receptor blockers. Undoubtedly, because the causes of pulmonary hypertension in the intensive care setting frequently are multifactorial, our “best” therapy will be multiply targeted. Adding phosphodiesterase inhibitors to prostacyclin infusions, endothelin blockers, thromboxane inhibitors, and inhaled nitric oxide (NO) all may have individual and combined merit with synergism enhancing efficacy.
NO is a selective pulmonary vasodilator that can be breathed as a gas and distributed across the alveoli to the pulmonary vascular smooth muscle.56 It is formed by the endothelium from L-arginine and molecular oxygen in a reaction catalyzed by NO synthase. It then diffuses to the adjacent vascular smooth muscle cells where it induces vasodilation through a cyclic guanosine monophosphate-dependent pathway.57 Because NO exists as a gas, it can be delivered by inhalation to the alveoli and then to the blood vessels, which lie in close proximity to ventilated lung. Because of its rapid inactivation by hemoglobin, inhaled NO may achieve selective pulmonary vasodilation when pulmonary vasoconstriction exists. It has advantages over intravenously administered vasodilators that cause systemic hypotension and increase intrapulmonary shunting. Inhaled NO lowers pulmonary artery pressure in a number of diseases without the unwanted effect of systemic hypotension. This effect is especially dramatic in children with cardiovascular disorders and postoperative patients with pulmonary hypertensive crises.50,58,59
Several groups have reported successful use of inhaled NO in a variety of other congenital heart defects after cardiac surgery. It may be especially helpful when administered during a pulmonary hypertensive crisis.59 NO use after Fontan procedures,60 after VSD repair, and with a variety of other anatomic lesions has been described. Prophylactic use of inhaled NO in patients at risk for developing postoperative pulmonary hypertensive crises is thought by some to reduce the duration of mechanical ventilation.61 Oxygen saturation in response to inhaled NO generally does not improve in very young infants who are excessively cyanotic after a bidirectional Glenn anastomosis. Increasing cardiac output and cerebral blood flow may have much greater impact on arterial oxygenation. Elevated pulmonary vascular tone is seldom the limiting factor in the hypoxemic patient after the bidirectional Glenn operation.62
Inhaled NO can be used diagnostically in neonates with RV hypertension after cardiac surgery to discern those with reversible vasoconstriction. Failure of the postoperative newborn with pulmonary hypertension to respond to NO successfully discriminated anatomic obstruction to pulmonary blood flow from pulmonary vasoconstriction. Failure of the postoperative newborn to respond to NO should be regarded as strong evidence of anatomic and possibly surgically remediable obstruction.63
If withdrawal of NO is necessary before resolution of the pathologic process, hemodynamic instability can be expected. The withdrawal response to inhaled NO can be attenuated by pretreatment with the type V phosphodiesterase inhibitor sildenafil.64 Sildenafil inhibits the inactivation of cyclic guanosine monophosphate within the vascular smooth muscle cell and has the potential to augment the effects of endogenous or exogenously administered NO to effect vascular smooth muscle relaxation. Sildenafil can be administered in an oral or IV form and has a somewhat selective pulmonary vasodilating capacity while lowering LA pressure and providing a modest degree of afterload reduction in some postoperative children. Chronic oral administration of sildenafil to adults with primary pulmonary hypertension improves exercise capacity, which suggests an important therapeutic application of the IV preparation in postoperative congenital heart surgery.
Cardiac Tamponade
In summary, aggressive identification and treatment of low cardiac output conditions after cardiac surgery is central to the critical care of children with CHD. Successful application of these strategies and thoughtful use of pharmacologic intervention undoubtedly has contributed to the remarkable decline in mortality associated with congenital heart surgery in the past two decades. However, despite these interventions, additional (mechanical) support is sometimes necessary as a bridge to recovery.
Mechanical Support of the Circulation
More than 300 children per year who receive ECMO for cardiac support are reported to the Extracorporeal Life Support Registry, with the majority of patients placed on ECMO after cardiotomy.65 Although more than 60% of these patients are decannulated from ECMO, the overall survival to discharge has been only 42% of reported cases.
Substantial institutional variability in patient selection for ECMO makes comparison of published experience difficult. Centers with an efficient and well-established ECMO service are more likely to use this form of support in patients with low cardiac output. Furthermore, surgical technique and bypass management are additional confounding factors that make comparisons of the use and indications for ECMO between institutions difficult to interpret. Nevertheless, this form of mechanical support can be demonstrated to be lifesaving, and it can be argued that it should be available when needed for selected patients after congenital heart surgery General indications and contraindications for ECMO support of the circulation in patients with CHD are summarized in Box 31-5 and Box 31-6.
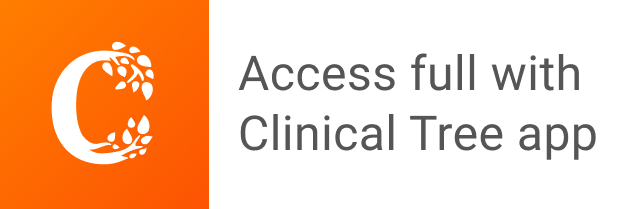