Pearls
- •
Serum creatinine is a crude assessment of filtration, influenced by several factors other than glomerular function, including gender, age, muscle mass, dilution, concentration relative to steady state, lack of baseline in many children, and testing modality. Changes in serum creatinine lag behind injury in many pediatric patients.
- •
Glomerular filtration estimation must be understood with precautions but may be more accurate using prediction formulas inclusive of muscle mass, kinetic changes, and consideration of other markers, such as cystatin C.
- •
Filtration and tubular function are distinct entities; urine output may be a more sensitive indicator of both epithelial tubular function and renal reserve.
- •
Use of glomerular filtration rate for assessment of kidney function must be adjudicated by physiology of the marker used and characteristics of the population in whom the equation was developed.
- •
Use of estimating equations in neonates must be taken in context of fetal age and maternal considerations.
- •
Next-generation methods of estimating kidney function will be multimodal, incorporating risk stratification, a combination of functional and damage biomarkers, integration of fluid accumulation, and assessment of renal reserve. Use of these methods will facilitate identification of acute kidney injury phenotypes.
Kidney dysfunction and injury are not unusual in the critically ill child and may develop as a consequence of the underlying disease process (e.g., sepsis, progressive glomerulonephritis) or its therapy (e.g., aminoglycoside toxicity). The ability to accurately, precisely, and rapidly assess kidney function is essential for the early detection of acute kidney injury (AKI), for dose adjustments of medications excreted by or toxic to the kidney, in risk assessment for imaging studies involving intravenous (IV) contrast agents or gadolinium, and for monitoring for medication-related nephrotoxicity. AKI is now recognized to encompass a spectrum of disease ranging from mild, reversible kidney dysfunction to severe, potentially irreversible organ failure with need for dialysis support. AKI occurs in a large population of critically ill children—1 in 4 requiring intensive care unit (ICU) treatment develop AKI in the first 7 days of the hospital course. In the past 10 to 15 years, standardized classification schemes for AKI based on an acute increase in serum creatinine or decrease in urine output (RIFLE [Risk, Injury, Failure, Loss, End-Stage Renal Disease]; AKIN [Acute Kidney Injury Network]; and KDIGO [Kidney Disease: Improving Global Outcomes]) have been proposed and validated in adults , , with modified versions proposed for children , ( Table 73.1 ). An increase in serum creatinine as small as 0.3 mg/dL is now well recognized to be associated with increased morbidity and mortality, underscoring the need for precise, sensitive, and timely measures of kidney function. , Notably, however, none of these definitions addresses AKI in the neonate. Jetton and Askenazi recently proposed a modification of the KDIGO classification for use in neonates ( eTable 73.2 ). Preterm infants frequently experience a serum creatinine elevation of 0.3 mg/dL; such increases can be associated with increased morbidity and mortality. The true incidence of AKI in the pediatric intensive care unit (PICU) is not well established, but recent studies suggest that it is increasing as advances in critical care medicine have led to improved patient survival and salvage of more critically ill individuals. , However, morbidity and mortality rates associated with AKI remain high. Therapeutic intervention trials for AKI have produced disappointing results, in part related to delays in diagnosis as well as the heterogeneity of patients enrolled. , Therefore, the focus is on earlier recognition and intervention for AKI, which should provide greater potential for recovery and/or stabilization of kidney function. However, the early detection of incipient AKI is severely limited by the lack of sensitive and specific tools currently available to assess kidney function.
Stage | Change in Serum Creatinine (S Cr ) | Urine Output |
---|---|---|
1 | ↑ ≥0.3 mg/dL over 48 h or ↑ 150%–200% over 7 days | <0.5 mL/kg/h for 8 h |
2 | ↑ ≥200%–300% | <0.5 mL/kg/h for 16 h |
3 | ↑ ≥300%, S Cr ≥4 mg/dL, or dialysis or eGFR ≤35 mL/min/1.73 m 2 for patients <18 y | <0.5 mL/kg/h for 24 h or anuria for 12 h |
Stage | Serum Creatinine (S Cr ) | Urine Output |
---|---|---|
0 | No change in S cr or ↑ ≤0.3 mg/dL | ≥0.5 mL/kg/h |
1 | S Cr ↑ ≥0.3 mg/dL within 48 h or ↑ ≥1.5–1.9 × reference S Cr a within 7 days | <0.5 mL/kg/h for 6–12 h |
2 | S Cr ↑ ≥2.0–2.9 × reference S Cr a | <0.5 mL/kg/h for ≥12 h |
3 | S Cr ↑ ≥3 × reference S Cr a or S Cr ≥2.5 mg/dL b or receipt of dialysis | <0.3 mL/kg/h for ≥24 h or anuria for ≥12 h |
a Baseline S Cr is defined as the lowest previous S cr value.
Assessment of glomerular function and injury
Glomerular filtration rate (GFR) is considered the best overall indicator of kidney function but remains challenging to accurately and efficiently measure in clinical practice. Functionally, the total kidney GFR is determined by the cumulative number of nephrons and the GFR within each nephron (single-nephron glomerular filtration rate [SNGFR]). Conceptually, it represents the volume of plasma that could be completely cleared of a substance per unit of time. Kidney function may decline due to hypoperfusion, resulting in a decrease in SNGFR, or due to nephron injury, resulting in fewer functioning nephrons; often both factors are present. The kidney has a certain degree of reserve and attempts to adapt to nephron loss by increasing the SNGFR of the remaining nephrons, thus maintaining total kidney GFR initially and masking early kidney injury. Indeed, by the time the GFR actually falls, significant injury has already occurred. Loss of this renal reserve is one of the earliest manifestations of kidney injury but is even more challenging to measure than the GFR. ,
Glomerular filtration is a dynamic variable that can fluctuate in a given individual by as much as 7% to 8% from day to day on the basis of differences in hydration status, activity, and protein consumption. The GFR is also influenced by age, gender, and body size; therefore, it varies between individuals as well. Thus, to facilitate comparison of the GFR among children and adults of considerably different sizes, the absolute GFR is normalized to body surface area (BSA), which correlates well with kidney weight, the most direct standard of reference. Appreciation of the maturational increase in GFR that occurs during infancy is also necessary for proper assessment of kidney function in children. At birth, the mean GFR is quite low (20 mL/min per 1.73 m 2 in term infants) due to renal immaturity not yet sufficient to meet the metabolic demands of a healthy infant. The GFR doubles within the first 2 weeks of life and then continues to gradually increase to reach a mean of 77 mL/min per 1.73 m 2 between 1 and 6 months of age and adult levels of 120 to 130 mL/min per 1.73 m 2 by approximately 2 years of age ( eTable 73.3 ).
Age | GFR (mL/min/1.73 m 2 ) | Range (mL/min/1.73 m 2 ) |
---|---|---|
Preterm (<34 wk) | ||
2–8 days | 11 | 11–15 |
4–28 days | 20 | 15–28 |
30–90 days | 50 | 40–65 |
Term (>34 wk) | ||
2–8 days | 39 | 17–60 |
4–28 days | 47 | 26–68 |
30–90 days | 58 | 30–86 |
1–6 mo | 77 | 39–114 |
6–12 mo | 103 | 49–157 |
12–19 mo | 127 | 62–191 |
2–12 y | 127 | 89–165 |
The GFR itself cannot be directly measured but can be assessed by measuring the clearance of an ideal filtration marker or estimated using predictive formulas. Urinary or plasma clearance studies provide the greatest accuracy but are expensive, time-consuming, and labor-intensive. Consequently, they are used mainly for research and in select clinical situations in which an accurate assessment of kidney function is necessary (e.g., chemotherapy dosing). For the daily clinical management of patients, serum creatinine and GFR estimating equations are used most commonly. They offer the advantage of being convenient, noninvasive, and inexpensive, with timely accessibility of results. However, accuracy and sensitivity to small changes in kidney function are sacrificed, making the detection of acute, early kidney dysfunction difficult. The search for novel biomarkers of kidney function (such as cystatin C) and early kidney injury (e.g., neutrophil gelatinase-associated lipocalin [NGAL]) has been a major focus in the field of AKI for this reason (see Biomarkers of Acute Kidney Injury and the Next Generation).
Significant barriers exist to practical and meaningful implementation of GFR estimation in critical care environments. Foremost, the majority of critically ill children do not have a baseline creatinine to use for comparison, either for absolute creatinine value change or change in GFR based on the formulas described earlier. Imputational methods have been derived and validated in numerous populations for the purpose of creating baseline creatinine values normative to age-, size-, or population-based GFR averages. Additionally, steady-state estimation for creatinine values are contextually skewed, as a high proportion of critically ill children are simply not at steady state on presentation and have total body water perturbations before, during, and after resuscitation. Pragmatic and innovative solutions are required, and are being developed, to modernize the approach to estimation of renal function in the critically ill child (see Biomarkers of Acute Kidney Injury and the Next Generation).
Renal clearance techniques
Classic, gold standard techniques for measuring the GFR using inulin, iothalamate, and creatinine clearance, and plasma disappearance techniques using radioisotopes are discussed at ExpertConsult.com .
Inulin
The renal clearance of inulin remains the gold standard for measuring GFR. Inulin is an inert, uncharged 5.2-kDa polymer of fructose that possesses many characteristics of an ideal filtration marker. It is not protein bound and is freely filtered at the glomerulus without being reabsorbed, secreted, or metabolized by the kidney. Further, it is eliminated exclusively by the kidney. Therefore, in the steady state, the filtered load of inulin (GFR × P in ) is equal to its urinary excretion (U in × V), where P in and U in are the plasma and urine concentrations of inulin (mg/dL), respectively, and V is the urine flow rate (mL/min). The renal clearance of inulin (C in ) can be calculated as follows:
Cin=GFR=(Uin×V)/Pin
C in is usually scaled for BSA:
Cin=GFR=[(Uin×V)/Pin]×[1.73m2/BSA]
The classic protocol for inulin clearance is cumbersome, involving an IV infusion of inulin over several hours and serial timed blood and urine specimens.
Iothalamate
Iothalamate (614 Da) is also freely filtered by the glomerulus and has been studied extensively as an alternative exogenous marker for GFR measurements. Unlike inulin, however, iothalamate has some protein binding (<8%) and proximal tubular secretion (10%), raising concern for overestimation of the GFR. Reported correlations between iothalamate and inulin renal clearances in the literature have been variable, with some studies demonstrating good correlation, whereas most suggest that iothalamate overestimates inulin clearance.
Creatinine clearance
Clearance studies that make use of endogenous markers such as serum creatinine obviate the need for a constant infusion and make the study more amenable to clinical practice. However, the problems associated with timed urine collections persist and, accordingly, limit the usefulness of this technique, particularly in children. Further, creatinine is a flawed filtration marker. Although it is predominantly eliminated by glomerular filtration, a small but variable amount (10%) is eliminated by tubular secretion. As renal function deteriorates, the proportion of secreted to filtered creatinine progressively increases, leading to an overestimation of GFR and a less reliable measure of GFR. Administration of oral cimetidine beginning 2 days before the study can at least partially circumvent this issue by inhibiting the tubular secretion of creatinine. Although timed urine collections are still necessary, they are typically obtained over a 2-hour period, facilitating performance in a monitored clinic setting.
Guidelines from the National Kidney Foundation no longer recommend routine performance of creatinine clearance studies to estimate GFR, as prediction formulas are considered more accurate. Nonetheless, creatinine clearance–based studies can still be helpful when assessing renal function in individuals with atypical body composition (e.g., anorexia, malnutrition) or dietary intake (e.g., vegetarian diet). ,
Plasma disappearance techniques
Plasma disappearance techniques further simplify the measurement of GFR and avoid the need for urine collections. They are most commonly performed using a single IV injection technique, although constant infusion and subcutaneous protocols are also available. , , Serial blood samples collected at specified times over a several-hour period following injection of the filtration marker are used to generate a plasma disappearance curve. This curve can be well approximated by a double exponential curve that is characterized by an initial “fast” curve and a later “slow” curve, as illustrated in eFig. 73.1 . The initial “fast” curve represents the distribution phase and reflects renal elimination as well as diffusion of the marker into the extravascular space, whereas the late “slow” curve reflects only the renal elimination phase. Renal clearance can be calculated by dividing the delivered dose by the entire area under the plasma disappearance curve. Modification from a two-compartment model to a one-compartment model focusing on the renal elimination phase simplifies the procedure, reducing the number of required blood specimens to two. The GFR can then be derived from the slope of the slow curve but requires incorporation of a correction factor to compensate for overestimation of the GFR, which results from exclusion of the area under the fast curve. Appropriate timing of the specimens is critical to ensure an accurate estimation of the GFR. The first sample must be obtained after the marker has equilibrated, typically 2 hours after injection. The second specimen is obtained approximately 5 hours after injection. However, in subjects with more advanced chronic kidney disease (CKD), this may need to be delayed further to minimize overestimation of the GFR, which can result from an inaccurate depiction of the lower slope of the plasma disappearance curve. The validity of the single-compartment model may also be compromised in edematous states in which the volume of distribution is increased or with extravasation of the tracer at the injection site, as this decreases the actual dose delivered. Although plasma disappearance studies are time-consuming and too complex for routine clinical care, they are useful in cases for which GFR estimates do not appear to be accurate.

Radioisotopes
Plasma clearance studies are most commonly performed with radioisotopes that are more readily available and easier to assay than inulin. Their major disadvantage is, of course, radiation exposure, which limits their use, particularly in children. 51 Cr-EDTA (chromium-51 ethylenediaminetetraacetic acid), 99m Tc-DTPA (technetium-99m diethylenetriaminepentaacetic acid), and 125 I-Iothalamate (iodine-125) have been extensively studied and compared with inulin renal clearance. , All are low-molecular-weight compounds that are freely filtered by the glomerulus but differ slightly in protein binding and renal disposition. 51 Cr-EDTA (292 Da) appears to have little protein binding, and its plasma clearance correlates well with inulin clearances. It is commonly used in Europe but unavailable in the United States. 99m Tc-DTPA (393 Da) is most frequently used in the United States but demonstrates variable accuracy in GFR measurements on the basis of product source, differences in protein binding, and potential dissociation of the chelate (t 1/2 = 6 hours) during the study. 125 I-Iothalamate (614 Da) is a high-osmolar anionic contrast agent with some protein binding that also undergoes active secretion by the proximal tubule, contributing to overestimation of plasma clearance compared with inulin or EDTA. Nuclear GFR studies can also be performed using a gamma camera, which measures the renal uptake of the tracer 2 to 3 minutes after injection and can provide information regarding differential kidney function. However, the estimated GFR obtained this way is not as accurate as with the plasma-sampling technique. To reduce radiation exposure, plasma clearance studies can also be performed using nonradiolabeled iothalamate, which can be measured by high-performance liquid chromatography (HPLC). ,
Iohexol
Iohexol is now widely accepted as an excellent alternative to inulin and radioisotopes for clearance studies. Iohexol (Omnipaque) is a low-molecular-weight (821 Da), nonionic, relatively low-osmolar IV contrast agent used routinely in the United States for radiologic studies at doses appreciably higher than that required for GFR studies. , It is almost entirely eliminated through glomerular filtration and is not reabsorbed, secreted, or metabolized by the kidney, thus fulfilling many of the desired traits of an ideal filtration marker. Further, it has minimal protein binding and negligible extrarenal elimination even in advanced chronic kidney failure. , It can easily be measured by HPLC or mass spectroscopy. , , Iohexol has been safely used for clearance studies for many years in Scandinavia , and the United States. , Iohexol plasma clearance results appear to be comparable with renal inulin clearance and plasma EDTA disappearance studies across a broad range of GFRs. , , Schwartz et al. , recently demonstrated the feasibility of conducting iohexol clearance studies using the finger prick technique with filter paper technology in adults, a procedure that would alleviate the need for repeated venipunctures or placement of a second IV for serial serum sample collection, making it attractive for use in children. However, additional studies are necessary before it is ready for widespread clinical use.
Renal inulin clearance compared with other glomerular filtration rate measurement techniques
Soveri et al. performed a comprehensive, systematic literature review examining the accuracy of GFR measurement techniques using renal inulin clearance as the gold standard. The renal clearance of EDTA and iothalamate, as well as the plasma disappearance of EDTA and iohexol, were considered suitable alternatives with an associated bias of less than 10% (mean difference between renal inulin clearance and that obtained using other techniques), P 30 greater than 80% and P 10 greater than 50% (percentage of GFR measurements within 30% and 10%, respectively, of inulin GFR). Creatinine clearance was not accurate, and there was insufficient data to accurately assess the use of the plasma clearance of DTPA.
Plasma markers
Creatinine
Serum creatinine is the most commonly used laboratory study to assess renal function in clinical practice. It is simple, convenient, and practical—requiring a single blood sample—and thus well suited for serial examination. However, the relationship between serum creatinine and GFR is quite complex, being influenced by several factors other than GFR. Therefore, at best, creatinine provides a crude estimate of the GFR. As illustrated in Fig. 73.2 , serum creatinine bears an inverse, nonlinear relationship with GFR, but it lacks sensitivity to acute and small changes in GFR. Notably, at low concentrations of serum creatinine corresponding to normal renal function, a substantial decrease in GFR may occur before being reflected by even a small increase in serum creatinine. In contrast, at higher levels of serum creatinine associated with renal failure, the same absolute rise in creatinine reflects a much smaller loss of remaining renal function. To a first approximation, every doubling of the serum creatinine represents a 50% decline in remaining GFR.

Ideally, an endogenous marker such as creatinine can serve as a useful surrogate for GFR if it is produced at a constant rate and eliminated only via the kidney at a rate equivalent to its production rate such that a steady state exists. The serum concentration of the marker would then be expected to rise with renal impairment. Serum creatinine, however, does not strictly fulfill these criteria. Although creatinine production is relatively constant, it varies among and within individuals. It is derived from the nonenzymatic dehydration of muscle creatine and hence is highly dependent on muscle mass. Consequently, in children, creatinine generation is affected by growth in addition to diet and illness, as seen with adults. The reference range for serum creatinine levels representing normal GFR will thus vary with age, size, and gender after puberty. Thus, the relationship between GFR and serum creatinine is particularly complex in children. Maturational changes in serum creatinine and GFR do not parallel one another. GFR is physiologically low at birth, whereas serum creatinine is elevated. However, because of fetal-maternal-placental equilibration of creatinine, the elevated creatinine is not indicative of the infant’s renal function but rather indicates the mother’s function. Following birth, the GFR steadily increases, reaching adult levels over the next 2 years. Serum creatinine, on the other hand, declines over the first few weeks, becoming reflective of the infant’s renal function. The creatinine level then remains stable until approximately 2 years of age as muscle mass accrues proportionally to the increase in GFR. Beyond 2 years of age, when GFR per BSA has fully matured, the ongoing accretion of muscle results in a progressive rise in serum creatinine until adolescence, when adult levels are achieved (0.7 mg/dL for adolescent females and 0.9 mg/dL for adolescent males). Superimposition of a severe or chronic illness associated with malnutrition and muscle wasting makes the interpretation of GFR from serum creatinine alone even more difficult in children. For example, at first glance, maintenance of a stable creatinine in a patient with a prolonged ICU course may be reassuring for preservation of renal function. However, if significant muscle atrophy has occurred, this actually suggests deterioration of renal function. In the steady state, when height is used as a surrogate for growth, there is a strong correlation of the parameter height/serum Cr (Ht/S Cr ) and GFR.
The second requirement, that excretion of the marker occurs only by glomerular filtration, is also flawed in the case of creatinine. Although the majority of serum creatinine is eliminated by glomerular filtration, a small but unpredictable amount is eliminated by tubular secretion and gastrointestinal degradation. Proximal tubular secretion of creatinine typically accounts for approximately 10% of its elimination, although considerable interindividual and intraindividual variability exist. At normal levels of GFR, the impact of tubular secretion on GFR is minimal. However, with deteriorating renal function, the proportion of secreted versus filtered creatinine increases, resulting in a lower serum creatinine than predicted for the true level of GFR, thus decreasing the sensitivity for serum creatinine to detect mild decreases in renal function. A similar phenomenon occurs in the setting of moderate-to-severe renal failure, when the bacterial degradation of creatinine within the gastrointestinal tract can become clinically significant, leading to a decrease in serum creatinine concentration. , Failure to recognize the influence of tubular secretion and gastrointestinal elimination on serum creatinine values can result in overestimation of renal function and may lead to higher, inappropriate dosing of medications. Conversely, in patients with advanced kidney injury, administration of medications (e.g., cimetidine, trimethoprim) that inhibit the tubular secretion of creatinine or administration of antibiotics that mitigate the gastrointestinal degradation of creatinine may result in an elevation of serum creatinine and subsequent underestimation of GFR without any true change in renal function. If not appreciated, this may be misconstrued as worsening renal function and lead to potential underdosing of medications. This issue is being addressed by integration of novel biomarkers that are capable of adjudicating and differentiating subclinical AKI, functional AKI, and damage-associated AKI (see Biomarkers of Acute Kidney Injury and the Next Generation).
Third, the requirement for a steady state cannot be overemphasized. When the GFR abruptly declines with AKI, the production rate of creatinine exceeds its clearance rate, leading to a gradual rise in serum creatinine that lags behind the true GFR. During this period, serum creatinine does not accurately reflect the true GFR. Nonetheless, serum creatinine is still typically used to estimate renal function, as better alternatives do not currently exist. However, use of the serum creatinine or creatinine-based estimating equations (presented later) to estimate GFR before the establishment of a new steady state will result in overestimation of renal function. Similarly, during the recovery phase of AKI, serum creatinine will underestimate the GFR as it will again lag behind the true GFR until the kidney clears the accumulated creatinine and reaches a new steady state.
Finally, analytic factors related to the creatinine assay itself provide another potential source of error when assessing GFR. True creatinine levels can be measured by isotope dilution mass spectroscopy (IDMS) or HPLC, but these methods are expensive and not readily available for routine clinical use. Enzymatic creatinine assays, now available in many laboratories, exhibit greater specificity for creatinine than the conventional Jaffe (alkaline picrate) assay, resulting in 10% to 30% lower creatinine concentrations. Enzymatic creatinine levels approximate HPLC values but can differ in their performance, some being more influenced by interfering substances than others. Although problems with accuracy and precision still persist, the coefficient of variation using new autoanalyzers is now approximately 3% and significantly lower than for the Jaffe assay. , Nonetheless, according to the College of American Pathologists in 2007, many laboratories continue to use a modified version of the Jaffe assay because it is less expensive than enzymatic assays. The Jaffe method, based on an alkaline picrate colorimetric reaction, tends to falsely elevate the true creatinine by up to 20% (for creatinine 1 mg/dL) owing to the presence of interfering, noncreatinine chromogens, the most significant of which is serum total protein. This effect is greatest at the lower levels of creatinine typically observed in infants and children. By falsely elevating the true creatinine, the Jaffe creatinine underestimates the GFR. Indeed, analytic variability is believed to play a greater role than biological variability in the day-to-day fluctuations of creatinine measurements. Speeckaert et al. published a correction accounting for the contribution of serum protein to Jaffe creatinine. When applied, this correction yields a creatinine value more consistent with that obtained using an enzymatic creatinine assay. An international effort to standardize creatinine measurements in clinical laboratories through use of a uniform assay and calibration materials was launched in the mid-2000s. , , All creatinine assays can now be calibrated to adult International Federation of Clinical Chemistry (IFCC) standards. However, the lowest IFCC standard is 1 mg/dL, which is still considerably higher than normal creatinine values for young children and those with decreased muscle mass.
Creatinine is affected by total body water. As a concentration-derived solute, the diluent volume of a host affects the resulting concentration regardless of assay used for measurement. Recent data describes clearly the change in AKI estimates based on creatinine before and after adjusting for total body water volume. Especially in pediatric patients, the change in total body water in relation to age is highly relevant and inversely related to change in creatinine concentration secondary to muscle mass development. Emerging data are required to further explore the concept of “corrected creatinine.” However, this adjustment is a practical and pragmatic reconciliation of the concentration of creatinine and total net fluid balance state (high accumulation deemed “fluid overload”). ,
Proper interpretation of the serum creatinine as a measure of GFR requires the physician to be knowledgeable about the clinical variables, physiologic processes, and analytic factors that can affect creatinine levels ( Table 73.4 ). Although the clinical laboratory provides normative reference ranges alongside the results, clinicians should confirm that the reported references are age appropriate and also realize that if the patient has decreased muscle mass (e.g., spina bifida, anorexia), even these age-appropriate reference ranges will be incorrect.
SERUM CREATININE LEVEL | ||
---|---|---|
Factor | Increase | Decrease |
Affecting creatinine generation | Age (infancy through adolescence) | Chronic illness; anorexia, malnutrition; neuromuscular disease (spina bifida, muscular dystrophy); liver disease |
Male gender (after puberty) | Body habitus (amputation) | |
Body habitus (muscular) | Diet (vegetarian) | |
Diet; consumption of cooked meat; creatine supplements | ||
Affecting creatinine elimination | Impaired tubular secretion (trimethoprim, cimetidine) | Tubular secretion |
Impaired extrarenal elimination (sterilization of gastrointestinal flora by antibiotics) | Extrarenal elimination (gastrointestinal degradation) |
Cystatin C
The well-established limitations of serum creatinine highlight the critical need to identify alternative biomarkers of renal function that have improved sensitivity and specificity. Some low-molecular-weight proteins have been considered potential candidate markers, as they are excreted primarily by glomerular filtration and are produced at a relatively constant rate. Several low-molecular-weight proteins have been considered but cystatin C, a 13-kDa cationic cysteine protease inhibitor produced at a constant rate by all nucleated cells, has shown the greatest promise. Like creatinine, it is freely filtered at the glomerulus but, unlike serum creatinine, it is not secreted by the tubules. Rather, it is completely reabsorbed and metabolized by the proximal tubule epithelial cells. In most studies, the production of cystatin C is not significantly affected by age, gender, and muscle mass, making it a particularly attractive marker in growing children, subjects with atypical body composition (e.g., malnutrition, anorexia, spina bifida, neuromuscular disease), and subjects experiencing rapid changes in muscle mass. Furthermore, in young children, the maturational changes associated with serum cystatin C follow those of the GFR better than serum creatinine. Serum cystatin C levels are elevated at birth, presumably due to the physiologically lower GFR. However, cystatin C, unlike creatinine, does not equilibrate across the placenta. Hence, the levels early after birth are more representative of the infant’s renal function than are creatinine levels and thus may facilitate the evaluation of renal function in newborns. Concurrent with the GFR increasing after birth, cystatin C levels decline to reach a plateau of 0.8 to 1.0 mg/dL by approximately 1.5 to 2.0 years of age. Beyond this age, serum cystatin C levels remain constant (until age 50 years), as does the GFR. Therefore, serum cystatin C levels may potentially facilitate the recognition of abnormal renal function, as growth is no longer a confounding variable. Furthermore, cystatin C has a shorter half-life than serum creatinine, making it more sensitive to acute and subtle changes in GFR. ,
In a small study of healthy subjects, serum cystatin C demonstrated less interindividual variability (25%) than serum creatinine (93%), suggesting that it may be a better marker for detecting the onset of acute renal dysfunction. However, the intraindividual variability greatly exceeded that of serum creatinine, limiting its usefulness to monitor the progression of CKD.
Studies comparing the use of cystatin C to serum creatinine as a diagnostic marker of kidney function have yielded conflicting results. Nonetheless, two meta-analyses, including adult and pediatric studies, suggest that cystatin C is superior or equivalent to serum creatinine as a marker of renal function. , Specifically, cystatin C may be advantageous in populations with low or atypical muscle mass (e.g., spina bifida) , and mild renal impairment. Changes in serum cystatin C levels may also potentially allow earlier detection of AKI. Herget-Rosenthal et al. found that cystatin C levels rose 1 to 2 days earlier than serum creatinine in critically ill adults with AKI. A meta-analysis in 2011 involving mainly adults concluded that serum cystatin C seems to be a good predictor of AKI. However, more recent studies in children have yielded conflicting results. , Further investigation regarding the use of cystatin C to predict and define AKI is clearly necessary.
Estimating equations
Empiric formulas (Cockcroft-Gault for adults, Schwartz and Counahan-Barratt for children) were first developed in the 1970s to enhance the physician’s ability to calculate an estimated GFR (eGFR) and, in particular, to facilitate the recognition of chronic renal impairment. This is especially helpful in children for whom the serum creatinine corresponding to normal kidney function progressively increases during childhood until adult levels are achieved during adolescence. The equations were developed in CKD populations and based on serum creatinine but incorporate clinical variables such as height, weight, age, and gender as surrogates for muscle mass. However, because they are creatinine based, they are subject to some of the same constraints as use of serum creatinine alone. For example, tubular creatinine secretion and technical issues related to the creatinine assay itself will influence eGFR results. Nonetheless, these equations perform better than serum creatinine alone. Current national guidelines now recommend that eGFRs be routinely reported alongside the creatinine value using the 2009 Chronic Kidney Disease Epidemiology Collaboration (CKD-EPI) formula for adults and the updated “bedside” Schwartz formula for children. Both formulas were developed using creatinine measurements calibrated to be traceable to an IDMS reference standard. Within the past decade, routine reporting of eGFRs has been implemented for adults but has been more challenging to implement for children because the equation is based on height, a parameter not routinely available in the laboratory electronic medical record. According to the College of American Pathologists, as of 2013, 90% of laboratories surveyed were reporting eGFRs along with creatinine for adults but less than 10% of laboratories surveyed were reporting eGFRs for children.
The ideal GFR estimating equation would be based on a standardized creatinine measurement, applicable across the full spectrum of renal function, and generalizable to a diverse population. In 2009, the CKD-EPI creatinine equation was developed using standardized creatinine measurements in a diverse population of adults with and without renal dysfunction. This was a substantial improvement over the 2006 Modification of Diet in Renal Disease (MDRD) equation, which was developed in adults with moderate CKD (GFR <60 mL/min per 1.73 m 2 ) and therefore systematically underestimated eGFR in patients with normal renal function or mild CKD. Limited studies assessing the application of these adult formulas in children have shown them to be inaccurate.
In pediatrics, the Schwartz formula has been most commonly used to predict GFRs. The original Schwartz formula was developed in the 1970s and based on a modified Jaffe creatinine, using inulin clearance as the reference standard. The GFR is related to serum creatinine, using length as a surrogate for muscle mass and an empiric constant to account for age- and gender-related differences in body composition:
eGFR=k×L/SCr
where eGFR is estimated GFR in mL/min per 1.73 m 2 , L is length in cm, S Cr is serum creatinine in mg/dL, and k is an empiric constant (i.e., 0.45 for term infants through the first year of life, 0.55 for children and adolescent females, and 0.7 for adolescent males). , The simplicity of the formula makes it convenient and practical for use at the bedside, although knowledge of the patient’s height is required. Counahan-Barratt developed a similar formula using 51 Cr-EDTA plasma disappearance as the reference standard. However, this formula uses a single constant with a lower value, attributed to a difference in creatinine assays:
eGFR(mL/min/1.73m2)=0.43×L/SCr
It is now well recognized that Schwartz’s original formula systematically overestimates GFRs, most likely due to a change in creatinine methodology over the years from the Jaffe assay to an IDMS-referenced enzymatic assay. As noted earlier, enzymatic creatinine levels in infants and children tend to run lower than Jaffe creatinine levels.
In 2009, using data from the Chronic Kidney Disease in Children Study (CKiD Study), Schwartz et al. developed an updated “bedside” formula on the basis of an IDMS traceable enzymatic creatinine assay using the plasma disappearance of iohexol as the reference standard:
eGFR=0.413×L/SCr
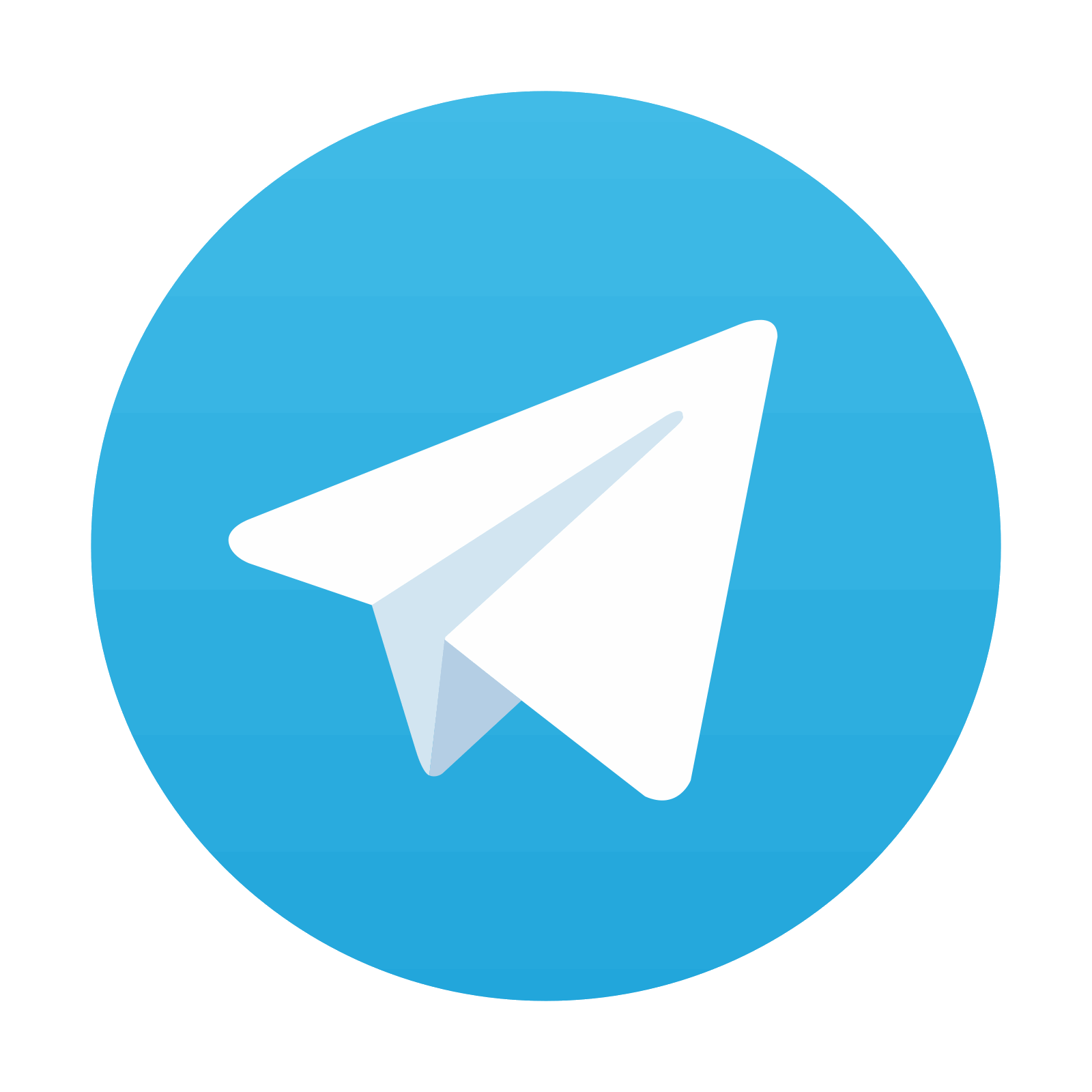
Stay updated, free articles. Join our Telegram channel
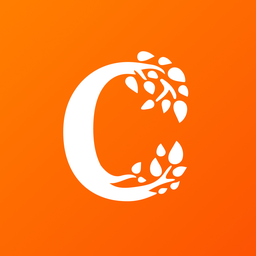
Full access? Get Clinical Tree
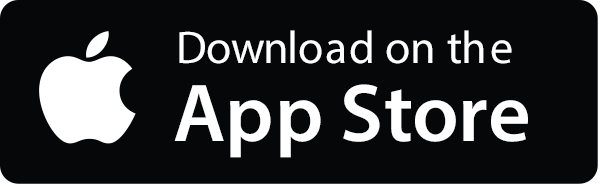
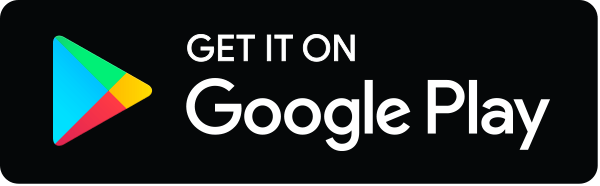
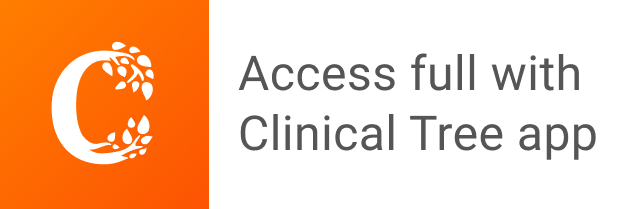