Pearls
- •
Children younger than 5 years have nearly equal peripheral and central airway resistance. This makes them more susceptible to developing respiratory failure with illnesses that affect the lower airway (such as bronchiolitis).
- •
In healthy adults, the critical closing capacity for most alveoli is much lower than functional residual capacity (FRC); thus, small losses in alveolar volume do not result in alveolar collapse. During infancy, the critical closing capacity for many alveoli lie above FRC, making infants prone to developing atelectasis and respiratory failure. Spontaneously breathing infants attempt to counteract this by stopping exhalation before reaching airway closure through laryngeal-breaking (sometimes termed auto-PEEP [positive end-expiratory pressure]), as an attempt to maintain a higher FRC.
- •
During airway obstruction, it is more efficient to breathe less often but with a higher tidal volume, as the resistance to airflow in the intrathoracic airways reduces with increasing volume. In contrast, when elastic work predominates (i.e., parenchymal lung disease), it is more efficient to breathe more often with lower tidal volume, as elastic work increases further as volume increases.
Physiology of the respiratory system
The respiratory system’s vital function is to enable gas exchange. The components responsible for effective gas exchange are complex and multifactorial. They comprise central nervous system control of respiratory drive and airway tone, the resistive properties of the upper and lower airways, elastic properties of the lung and chest wall, diffusion across the alveolar-capillary membrane, transport of gases in the blood, diffusion of gases into the cells of the body, and the use of oxygen and production of carbon dioxide (CO 2 ) within cells as a by-product of metabolism.
This chapter introduces key concepts to better understand how they become deranged during critical illness and are modified by therapies applied in intensive care. To facilitate understanding of these concepts, a compartment-based model of the respiratory system is presented. This framework is used to highlight pieces of respiratory physiology most relevant for pediatric intensive care practitioners.
There are four main respiratory compartments: (1) central nervous system control; (2) extrathoracic upper airways; (3) the intrathoracic compartment, which includes the lower airways and pulmonary parenchyma; and (4) the extrathoracic space, including the chest wall and abdomen. Important principles of gas exchange are also covered in this chapter. Some concepts are relevant to multiple topics covered in this text; we hope that this framework facilitates linking physiologic concepts with clinical care. An introduction to a few important principles is therefore presented first.
Boyle’s law
The first step of gas exchange is alveolar ventilation, which requires movement of air in and out of the lungs. This movement is driven by Boyle’s law, which states that within a structure, there is an inverse relationship between pressure and volume such that a rise in volume results in a fall in pressure or vice versa. During normal breathing, the diaphragm contracts and is displaced downward. This expands the thoracic cavity and generates negative pleural and airway pressure. Because the airway pressure is negative relative to the atmosphere, the pressure gradient causes air to flow from the atmosphere into the lungs. During exhalation, elastin fibers in the alveoli recoil and the respiratory muscles relax, causing the volume of the thoracic cavity to decrease. This makes the pressure inside the airways more positive relative to the atmosphere, causing air to flow from the airways back out into the atmosphere.
Equation of motion of the respiratory system
Motion of gas within the respiratory system requires force to overcome the flow-resistive, inertial, and elastic properties of the airways, lung parenchyma, and chest wall. Under normal circumstances, this force is generated by the respiratory muscles. At each instant, the applied pressure in the respiratory system (P RS ) must equal the sum of the pressure required to overcome elastic recoil (P ER ) and the pressure lost to viscous (resistive) forces (P R ).
P ER is a function of the elastance of the respiratory system (1/compliance) and the change in lung volume during a respiratory cycle (i.e., tidal volume [V]). P R is a function of the resistance in the airways and the flow (Q). This is typically expressed as the equation of motion of the respiratory system :
Under circumstances of unassisted breathing, P RS is generated entirely by the respiratory muscles and is referred to as P MUS . However, if the patient is receiving positive pressure ventilation, some or all of this pressure is shared between the patient and the ventilator. Decreases in respiratory system compliance (or increases in respiratory system elastance, such as from alveolar edema or consolidation) or increases in airway resistance (i.e., from bronchoconstriction or upper airway obstruction) will result in an increase in P RS . During unassisted breathing, this manifests as larger patient effort needed to generate this pressure; during controlled mechanical ventilation, it results in higher delta pressure (peak inspiratory pressure [PIP] – positive end expiratory pressure [PEEP]) on the ventilator.
Transmural pressures
While the equation of motion of the respiratory system relates to the net pressure gradient for gas to flow into the lungs, there are individual pressure gradients across the various components of the respiratory system that drive either the rate of gas flow or the expansion of volume of the tissue ( Fig. 42.1 ). The first is the airway pressure gradient, which drives airflow from the atmosphere into the alveoli and is defined as:

where P bs is the pressure at the body surface (or mouth), which is normally atmospheric, and P ALV is the alveolar pressure. Larger reductions in alveolar pressure during inspiration result in more airflow.
The remaining pressures are typically measured during static conditions (no airflow), as they provide information about elastic recoil properties of the lung, chest wall, and the entire respiratory system. At the alveolar level, the important pressure gradient is expressed as the transpulmonary pressure (P TP ), defined as
where P ALV is the alveolar pressure and P pl is the pleural pressure. P TP is equal to elastic recoil of the lungs when there is no airflow. During healthy conditions, P TP is typically slightly positive (to keep alveoli expanded) at end exhalation and becomes more positive with increasing lung volume (such as during tidal ventilation).
Transchest wall pressure (P TC ) is defined as
where P pl is the pleural pressure and P bs is the pressure at the body surface that is usually atmospheric (i.e., 0). P TC and P pl represent the elastic recoil of the chest when there is no airflow and, like P TP , they increase and decrease with lung volume.
Finally, the transmural pressure across the respiratory system (P RS ; lung and chest wall) is defined as
where P ALV is the alveolar pressure and P bs is the pressure at the body surface. P RS is equal to the net passive elastic recoil pressure of the whole respiratory system when airflow is zero.
Central nervous system control
Respiratory centers in the brainstem set the rhythm for normal breathing, transmitted through efferent nerve pathways that terminate at the respiratory muscles. Afferent nerve pathways send information back to the respiratory centers in the brainstem on the adequacy of gas exchange (chemoreceptors), the state of lung inflation (mechanoreceptors), and pathologic disturbances (nociceptors). Based on this feedback, respiratory centers alter the amplitude and rate of efferent nerve impulses being sent to the respiratory muscles. By using this feedback loop, a stable amount of oxygen and carbon dioxide can be maintained at the cellular level.
Respiratory centers and efferent nerve transmission to the respiratory muscles
The respiratory centers are housed within the medulla and pons, in the ventral respiratory group of the ventrolateral brainstem. The ventral respiratory group is a collection of three nuclear groups (the pre-Botzinger complex, nucleus ambiguous, and the nucleus retroambigualis). These neurons are considered upper motor neurons of the respiratory system. They innervate motor neurons in the anterior horns of the spinal cord and cause contraction in muscles contained in the upper airway, the diaphragm, and the intercostals. Derangement in these respiratory control centers from congenital lesions (e.g., central hypoventilation syndrome), acquired conditions (e.g., acute or chronic forms of encephalopathy), or therapies (e.g., sedation and anesthesia) are common in critical care and may contribute to respiratory failure in children.
Receptors and feedback to the respiratory centers
Chemoreceptors sense the concentration of oxygen, CO 2 , and hydrogen (H + ) ions at various locations within the central and peripheral nervous systems. The central chemoreceptors are located near the ventral-lateral surface of the medulla, in contact with the cerebrospinal fluid (CSF). As arterial CO 2 content increases, more CO 2 diffuses into the CSF and binds with water to release H + , resulting in stimulation of these central chemoreceptors. The parafacial retrotrapezoid nucleus (RTN), which contains Phox2b-expressing glutamatergic neurons, has been identified as a likely location for the chemoreceptors that sense CO 2 in the central nervous system, as a mutation in Phox2b is a risk factor for developing congenital hypoventilation syndrome, where severe hypoventilation occurs during sleep or sedation. , The peripheral chemoreceptors consist of the carotid bodies located at the bifurcation of the common carotid artery and aortic bodies located in the aortic arch. The carotid bodies are the primary oxygen chemoreceptor. They become activated when partial pressure of arterial oxygen (Pa o 2 ) falls below 100 mm Hg and can be modulated further by changes in CO 2 and H + in arterial blood. The aortic bodies respond to reductions in arterial pH. There is a link between central and peripheral chemoreceptors with Phox2b neurons, which consequently increases or decreases the slope of the central CO 2 ventilatory response when the peripheral chemoreceptors are stimulated.
Pulmonary mechanoreceptors sense the adequacy of lung stretch and send afferent messages back to the respiratory centers. Within the tracheobronchial superficial mucosal layer are rapidly adapting pulmonary stretch receptors that when activated result in elevated airway resistance, reflex apnea, and coughing. Within the tracheobronchial smooth muscle layer are the slowly adapting pulmonary stretch receptors that when activated send messages through the vagal afferent myelinated fibers to terminate inspiration. This reflex to end inspiration, which is driven by these pulmonary stretch-sensitive mechanoreceptors, is termed the Hering-Breuer inflation reflex . This alone can relieve feelings of dyspnea from unsatisfied inspiration independent of arterial blood gas concentration.
Receptors that sense injury to tissues (nociceptors) exist in the respiratory system. In the upper respiratory tract, irritants on the mucosal surface triggered by mechanical touch can initiate a rapid cough. In the lower respiratory tract, bronchopulmonary C-fibers, which are slower-acting unmyelinated fibers housed near the pulmonary interstitium, are activated by inflammation and can trigger defensive mechanisms such as apnea, rapid shallow breathing, mucus secretion, bronchoconstriction, and cough. ,
Higher states
Level of consciousness can further modulate respiratory drive. In the sleeping or obtunded patient, control is dominated by chemoreceptor feedback. Chemoreception with the RTN manages a large portion of the drive to breathe during non–rapid eye movement (REM) sleep; during REM sleep, the contribution of the RTN is still present but affects only some components of breathing. During wakefulness and non-REM sleep, the RTN regulates both respiratory rate and tidal volume. During REM sleep, however, the RTN regulates only tidal volume.
Opioid drugs, such as morphine and fentanyl, reduce motor control and attention, which can alter sleep. Respiratory rhythm generation is highly influenced by the pre-Botzinger complex in the ventrolateral medulla; this site is greatly inhibited by opioids, which reduce respiratory rate without a significant change in tidal volume (although this can also occur during high doses).
Volatile anesthetics and propofol can suppress breathing by blunting chemoreception, inhibiting motor neurons, and by eliminating the wakefulness state. During anesthesia, the response to hypoxic drive is blunted even prior to a loss of consciousness. During sleep, respiratory rate is left unchanged or increased while the tidal volume and response to oxygen chemoreception is decreased.
Extrathoracic upper airways
When evaluating the extrathoracic upper airways, the physiology and pathophysiology typically follow airway anatomy. Therefore, it is useful to compartmentalize the upper airway into supraglottic regions (i.e., the nasopharynx and oropharynx), the region near the glottis (i.e., vocal cords and folds), and subglottic region (i.e., the extrathoracic trachea). Given the anatomic considerations of the pediatric airway—as well as physiologic concepts relating the relationship between airway caliber, resistance, and flow—children are particularly prone to develop respiratory failure related to upper airway obstruction from lesions in any one of the three extrathoracic compartments.
Anatomic considerations for the pediatric upper airway
Anatomically, the configuration of the airway changes considerably as a child develops from infancy to adulthood. The infant’s airway is characterized by a relatively small mandible and flat roof of the nasopharynx. As the child develops, the mandible descends and moves forward, and the roof of the pharynx becomes more rounded. Moreover, the tongue is relatively large compared to the bony structures in the mouth, and the normal anterior displacement of the tongue with inspiration is relatively blunted in the young infant. As a result, loss of airway tone from sedation, sleep, or CNS dysfunction is more likely to result in obstruction of the posterior pharynx in a young child than in an adult.
Compared with the adult, the infant’s larynx is high in the neck; at birth, the epiglottis often overlaps the soft palate at the level of the first cervical vertebrae. The epiglottis separates from the soft palate by approximately 6 months of age and descends to the third cervical vertebrae. It reaches the adult position, at the fifth or sixth cervical vertebrae, by adolescence. The infant’s epiglottis is rounder and softer than the adult epiglottis and is more prone to occlude the airway. Finally, it has been a longstanding belief that the airway of an infant tapers in a funnel shape and becomes narrowest at the cricoid cartilage, in the subglottic space, and it remains this way until about 8 to 10 years of age. However, more recent evidence that has examined computed tomography scans and ultrasound of pediatric patients questions some of these findings. Nevertheless, the area at highest risk for injury from endotracheal intubation does appear to be below the vocal cords, in the subglottic space.
Ohm’s law and Poiseuille’s law
Air moving through the upper airway can be modeled as a fluid moving through a pipe. The rate of flow through a tube is a function of the resistance and pressure gradient, which can be modified from Ohm’s law, where voltage (V) equals the current (I) multiplied by resistance (R). In physiologic considerations of airflow, V represents the pressure gradient across the system (ΔP), and I represents the flow (Q), yielding the relationship Q = ΔP /R. The resistance in the airway can be modeled from Poiseuille’s law, where length (l) of the pipe, its radius (r), and the viscosity of gas (η) affect the resistance (R = 8ηl/πr 4 ). Since the radius term is in the denominator and is raised to the fourth power, the effect of an internal radius reduction of one-half can increase the resistance by 16-fold under laminar conditions and even more so during turbulent flow. Since the internal lumen of the pediatric airway is much smaller than an adult airway, the resistance to flow is already exponentially higher. Further reductions in the cross-sectional area of the airway (such as from croup) lead to an even more significant increase in airway resistance.
Turbulent versus laminar flow (Reynolds number)
When flow is turbulent rather than laminar, the relationship between pressure and flow is no longer linear, making the resistance more difficult to estimate directly. Nevertheless, most believe that a modification of Poiseuille’s law can be applied, but the radius term in the denominator is now raised to the fifth power instead of the fourth, making the impact of airway caliber even more critical during conditions of turbulent flow. Laminar versus turbulent flow is primarily determined from the Reynolds number (Re) which is given by
where d is the diameter, v is the mean velocity, ƥ is the density of the gas, and μ is the viscosity. Higher Reynolds numbers (>4000) result in turbulent flow whereas lower numbers (<2000) result in laminar flow, with a point of transition in between. Hence, conditions that increase mean velocity (i.e., increased flow), increase the density of the gas, or decrease the viscosity will promote turbulent flow. This is the physiologic principle behind heliox, as replacing nitrogen with helium—a gas of much lower density but similar viscosity—reduces the Reynolds number.
Transmural pressure (collapse of upper airway)
Airway caliber changes dynamically throughout the respiratory cycle because of changes in transmural pressures. Transmural pressure is defined as the pressure inside a structure minus the pressure outside. When considering the upper airway, negative pressure during inspiration within the airway relative to atmospheric pressure outside the airway will result in a slightly negative transmural pressure and a tendency of the airway walls to collapse inward. This reverses during exhalation, with pressure within the airway being slightly positive relative to the atmospheric pressure. The degree of airway caliber change during inspiration depends on both the magnitude of the transmural pressure and airway wall compliance. During normal conditions, airway caliber changes minimally. However, as airway pressure becomes more negative (i.e., in an attempt to overcome an area of increased airway resistance), airway caliber is further reduced at the point distal to the obstruction. This is classically seen in cases such as viral croup, in which there is further dynamic collapse of the upper airway typically just below the area of inflammation ( Fig. 42.2 ). The opposite occurs during exhalation, which is typically why croup manifests predominantly with inspiratory stridor.

Inspiratory flow limitation
When inspiration and expiration are unobstructed, flow steadily increases during inspiration, reaches peak flow, and begins to decelerate as it approaches zero just before exhalation begins. Volume increases with this flow, giving rise to the classic appearance of a flow-volume loop ( Fig. 42.3 A). However, this pattern is altered in the presence of upper airway obstruction. In circumstances of extrathoracic, dynamic obstruction (such as croup), the flow-volume loop shows flattening of inspiratory flow over a large range of volume with inspiratory flow limitation ( Fig. 42.3 B), where no further increase in flow is possible due to the lesion itself in combination with dynamic airway collapse. Truly identifying inspiratory flow limitation mandates knowing that patient effort is continuing, but flow cannot be increased. Thus, if one combines a measure of patient effort—such as an esophageal catheter that provides a surrogate for pleural pressure—with a measure of flow, one can see no rise (or minimal rise) in inspiratory flow with significant patient effort ( Fig. 42.3 C–D). This appears as a flat portion on the top of the flow-pressure plot.

Furthermore, one can also pinpoint the location of the obstruction by evaluating whether changes in airway positioning improve flow limitation. In general, supraglottic lesions respond to changes in airway position, such as a jaw thrust (Esmarch maneuver). In circumstances of poor airway wall compliance (such as subglottic stenosis or complete tracheal rings), there is less dynamic collapse of the upper airway during inspiration. Here there is both inspiratory and expiratory flow limitation, with minimal change in airway caliber from inspiration to exhalation.
Intrathoracic compartment
Flow resistance of the respiratory system
The response of the lung to movement is governed by the physical impedance of the respiratory system. The impedance can be categorized into (1) elastic resistance between the alveolar gas/liquid interface and tissue, and (2) frictional resistance to gas flow. Under static conditions (no flow), pressure is required only to oppose the elastic recoil of the respiratory system. However, when the lungs and chest wall are in motion and movement of air into and out of the lungs occurs, pressure also must be provided to overcome the frictional or viscous forces. The ratio of this additional pressure (P) and the rate of airflow (Q) that it produces is defined as the resistance:
In other words, the flow (Q) measured at the mouth depends on the change in pressure across the respiratory system (i.e., the pressure difference between alveoli [P alv ] and mouth [P mo ] during spontaneous breathing) and the airway resistance (R aw ):
R aw is the sum of the peripheral airway resistance (peripheral intrathoracic airways <2 mm diameter [R awp ]), the central airway resistance (large intrathoracic airways >2 mm diameter [R awc ]), and the extrathoracic airway resistance (especially glottis [R ext ]). In healthy people, R ext accounts for 50% of the total R aw and R awp for about 15%, although this relationship fluctuates as a function of age as more peripheral airways develop into adulthood. In fact, peripheral and central airway resistance is nearly equal for infants and toddlers, with a fall in peripheral airway resistance typically around 5 years of age.
While airway resistance is most commonly altered in disease states, total respiratory resistance (R rs ) consists of the resistance of the airways (R aw ), resistance of the lung (R L ), and resistance of the chest wall (R cw ):
In older children, R cw and R L represent only 10% to 20% of R rs . However, in newborns, R L could be higher. During quiet breathing, R aw accounts for greater than 50% of the total respiratory system resistance. As flow increases, airway resistance contributes further to total respiratory system resistance. Increased flow can also result in nonlinear flow-resistance characteristics that lead to progressively more turbulent flow and therefore larger increases in airway resistance, as discussed in the upper airway compartment section. For patients who are breathing quietly by mouth, total airway resistance is divided almost equally between the upper and lower airways. As flow rate increases, the ratio of upper to lower airway resistance progressively increases as well.
There is also a volume dependency for R aw because higher lung volumes give higher elastic recoil properties of the chest wall (P el ) and therefore increase airway diameter, which decreases R aw . The specific relationship between R aw (or its reciprocal conductance G aw [= 1/R aw ]) and volume is mirrored by the specific R aw (sR aw ) and specific G aw (sG aw ):
The resistance of the airways (R aw ), lungs (airway and parenchyma [R L ]), chest wall (R cw ), and entire respiratory system (R RS ) can be calculated by measuring the rate of airflow and associated transstructural pressure by subtracting from the total pressure the amount required to overcome elastic recoil:
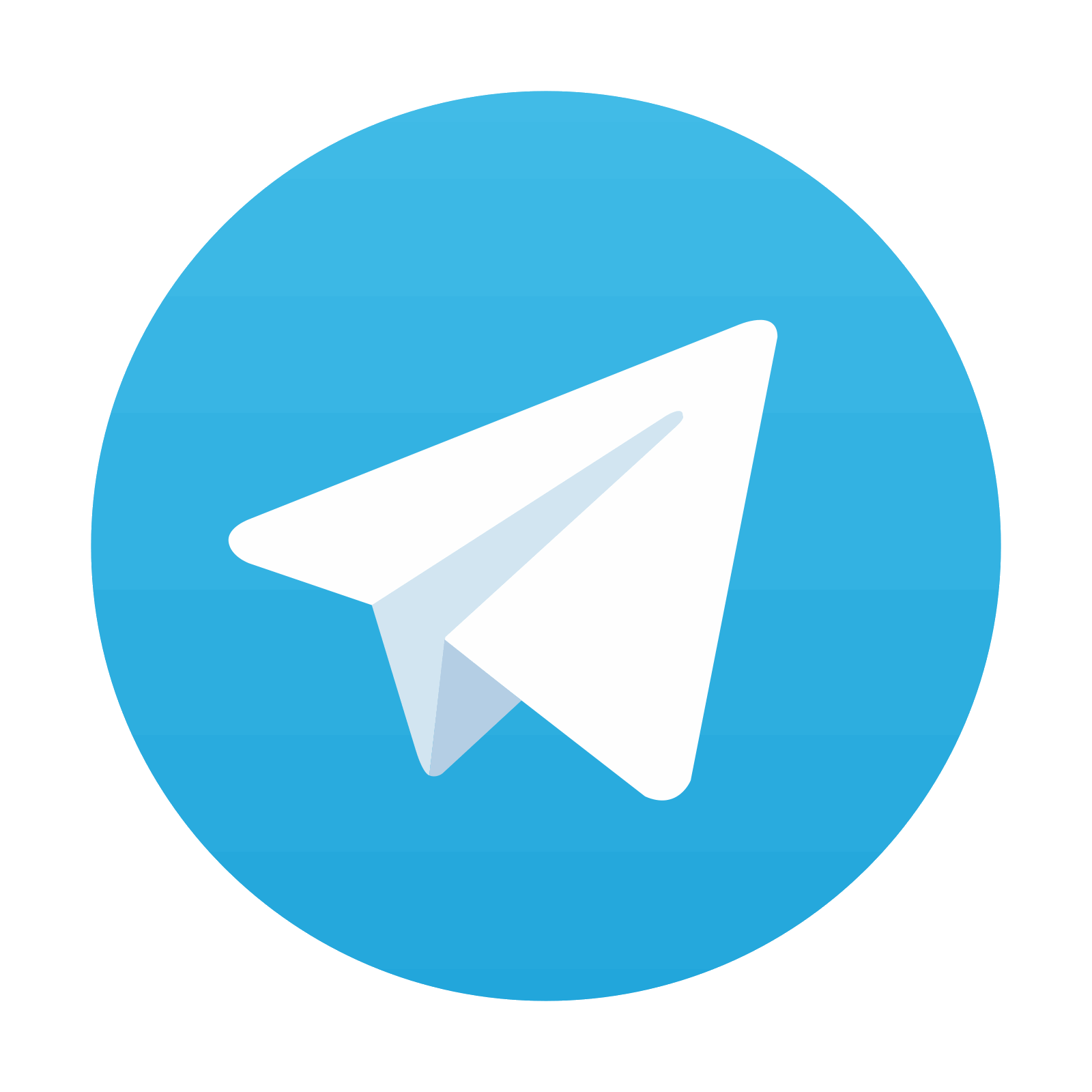
Stay updated, free articles. Join our Telegram channel
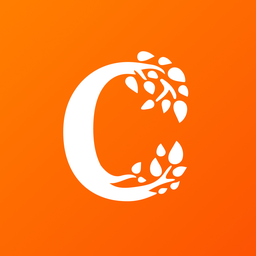
Full access? Get Clinical Tree
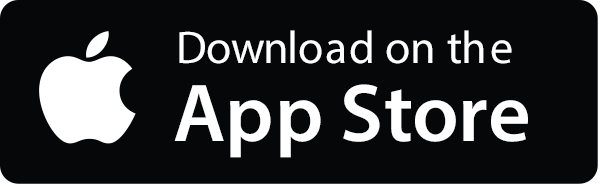
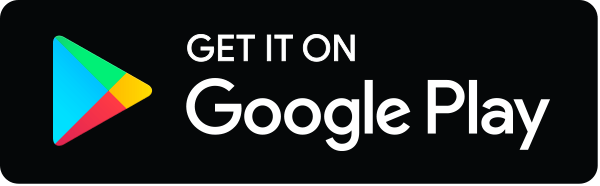
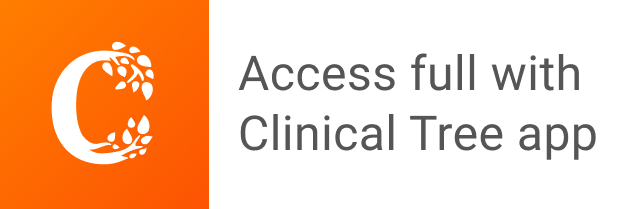