Setting the Ventilator: Introduction
The choice of ventilator settings should be guided by clearly defined therapeutic end points. In most instances, the primary goal of mechanical ventilation is to correct abnormalities in arterial blood-gas tensions. In most patients, this is accomplished easily by adjusting the minute volume to correct hypercapnia and by treating hypoxemia with oxygen (O2) supplementation. Because the volume, frequency, and timing of gas delivered to the lungs have important disease-specific effects on cardiovascular and respiratory systems functions, the physician must avoid simply managing the blood-gas tensions of the ventilator-dependent patient. After a brief review of the capabilities of modern ventilators, this chapter discusses the mechanical determinants of patient–ventilator interactions and defines therapeutic end points in common respiratory failure syndromes. These sections provide background for the major thrust of the chapter, which is to detail the physiologic consequences of positive-pressure ventilation and to develop recommendations for ventilator settings in various disease states based on this knowledge.
Capabilities of Modern Ventilators
The incorporation of microprocessors into ventilator technology has made it possible to program ventilators to deliver gas with virtually any pressure or flow profile. Significant advances have been made in producing machines that are more responsive to changes in patient ventilatory demands, and most full-service mechanical ventilators display diagnostic information contained in airway pressure (Paw), volume (V), and flow () waveforms. Because of these added capabilities, the practitioner is being challenged with a staggering array of descriptive acronyms for so-called new modes of ventilation. To avoid unnecessary confusion, it is useful not to focus on specific modes for the moment but rather to consider three general aspects of ventilator management: (a) the choice of inspired-gas composition, (b) the means to ensure the machine’s sensing of the patient’s demand, and (c) the definition of the machine’s mechanical output.
Practically speaking, decisions regarding the composition of inspired gas concern only the O2 concentration (see “Acute Lung Injury and Hypoxic Respiratory Failure” below). Although there may be occasions when the care provider considers supplementing the inspired gas with nitric oxide, the efficacy of nitric oxide therapy for most forms of hypoxic respiratory failure remains to be established.1 There has been growing interest in the biologic effects of hypercapnia on gas exchange, vascular barrier properties, and innate immunity.2–7 Therapeutic hypercapnia, that is, the deliberate supplementation of inspired gas with carbon dioxide (CO2), however, cannot be recommended at this point in time. On extremely rare occasions, it may be appropriate to use a helium-oxygen mixture in an attempt to lower the flow resistance across a lesion in the distal trachea or mainstem bronchi, and there has been some interest in the use of helium in asthma.8 Currently, these approaches must be considered experimental.
Ideally, a mechanical ventilator should adjust not only its rate but also its instantaneous mechanical output in response to changing patient demands. Conventional modes of ventilation cannot do so; instead, such modes execute a predefined pressure or flow program after an effort has been sensed.
Volume preset controlled mechanical ventilation (CMV) refers to a mode during which rate, tidal volume (VT), inspiratory-to-expiratory timing (I:E ratio), and inspiratory flow profile are determined entirely by machine settings and cannot be altered by either the rate nor the amplitude of the patient’s effort. Occasionally, investigators refer to ventilation as “controlled” when spontaneous respiratory muscle activity has been abolished by mechanical hyperventilation or by pharmacologic means (e.g., sedation and neuromuscular blockade).
Assist-control ventilation (ACV) gives the patient the option of initiating additional machine breaths when the rate, set by the physician, is insufficient to meet the patient’s rate demand. ACV differs from intermittent mandatory ventilation (IMV) in that all delivered breaths execute the same pressure or flow program, depending on the choice of primary mode. The ACV feature has lured many providers into the erroneous assumption that the primary machine rate setting is unimportant (see “Acute Lung Injury and Hypoxic Respiratory Failure: Respiratory Rate” below).
Traditionally, machine algorithms for detecting patient effort have keyed on the airway pressure signal.9 Because the inspiratory port of ventilators is closed during machine expiration, any inspiratory effort that is initiated near relaxation volume (Vrel) causes a fall in Paw. When Paw reaches a predefined trigger threshold (usually set 1 to 2 cm H2O below the end-expiratory pressure setting), the machine switches from expiration to inspiration. In the presence of dynamic hyperinflation, the inspiratory muscles must generate considerably more pressure than the set airway trigger pressure before a machine breath is delivered10 (see “Obstructive Lung Diseases” below).
Particularly in older ventilator models and in less-sophisticated portable machines intended for home use, it used to be common to find delays of up to 0.5 second between the onset of inspiratory muscle activity and machine response. In most ventilators used today, such delays are less than 100 milliseconds.11 Sensing delays are common when the Paw is monitored in the machine rather than near the patient–ventilator interphase. In the former case, the ventilator tubing acts as a capacitor, delaying the transmission of pressure from the intrathoracic airway to the pressure transducer. Additional delays can be attributed to dynamic hyperinflation and physical constraints on the opening and closing of demands valves. Considering that most ventilator-dependent patients generate between 4 and 8 cm H2O pressure in 100 milliseconds,10,12 delays can cause significant effort expenditure and discomfort. More importantly, patients may terminate seemingly ineffective inspiratory efforts prematurely only to initiate another effort of greater amplitude shortly thereafter. This leads to discrepancies between patient and machine rate.13,14 Discrepancies are seen often in weak or heavily sedated patients with severe hyperinflation and high intrinsic respiratory rates.13,15
Flow-triggering algorithms are alternatives or adjuncts to Paw-based triggering. During “flow triggering,” a base flow of gas is being delivered to the patient during the expiratory as well as the inspiratory phases of the machine cycle.9 Unless the patient makes an inspiratory effort, gas bypasses the endotracheal tube and is discarded through the expiratory machine port. In the absence of patient effort, expiratory flow is equal to inspiratory base flow. In the presence of an inspiratory effort, gas enters the patient’s lungs and is thereby diverted from the expiratory machine port. A discrepancy between inspiratory and expiratory base flow is sensed, and the ventilator switches phase. Because “flow triggering” alleviates the need to rarefy gas against an occluded demand valve, initially it was considered superior to pressure-based trigger algorithms.9,16 Because most new-generation ventilators have combined pressure and flow-sensing capabilities, these distinctions no longer apply.
The mode of mechanical ventilation often refers to the shape of the inspiratory pressure or flow profile and determines whether a patient can augment VT or rate through his or her own efforts.
In conventional volume-preset mode, each machine breath is delivered with the same predefined inspiratory flow-time profile. Because the area under a flow-time curve defines volume, VT remains fixed and is uninfluenced by the patient’s effort. Volume-preset ventilation with constant (square wave) or decelerating inspiratory flow is the most widely used breath-delivery mode. Breath delivery with flows that decrease with increasing lung volume are effective in reducing peak Paw. It is not clear, however, whether they protect the lungs from overdistension injury any more than square wave flow profiles.
The mechanical output of a ventilator operating in the volume-preset mode is uniquely defined by four settings: (a) the shape of the inspiratory flow profile, (b) VT, (c) machine rate, and (d) a timing variable in the form of either the I:E ratio, the duty cycle (TI/TTOT [inspiratory time/total respiratory time]), or the TI. In some ventilators, timing is set indirectly through the choice of peak or mean inspiratory flow (VT/TI). Figure 5-1 illustrates the relationships among these and other breathing-pattern parameters of significance.
Figure 5-1
Idealized spirogram of a breath delivered during volume-preset mechanical ventilation. Examples 1 through 6 indicate specific changes in ventilator settings and illustrate the consequences on flow and timing variable. (For abbreviations, see Table 5-1.) (1) Increasing mean inspiratory flow (VT/TI) at a constant machine rate setting results in a reduced I:E ratio and vice versa. TI, TI/TTOT, and mean expiratory flow (VT/TE) decline. (2) Increasing VT at constant TI/TTOT or I:E setting increases mean inspiratory flow and requires an increase in mean expiratory flow. Remember that mean inspiratory flow equals peak inspiratory flow when delivery modes with constant square wave flow profiles are used. (3) Increasing VT at a constant mean inspiratory flow setting increases TI, TI/TTOT, I:E ratio, and mean expiratory flow. (4) Decreasing mean inspiratory flow at a constant machine rate setting results in an increase in the I:E ratio and vice versa. TI, TI/TTOT and mean expiratory flow rise. (5) Reducing the machine backup rate (fM) at a fixed I:E ratio or TI/TTOT setting always prolongs TI and lowers mean inspiratory flow. The timing effects of reducing fM at a fixed inspiratory-flow setting cannot be predicted without knowledge of the patient’s actual trigger rate. (6) Increasing fM at a fixed I:E ratio or TI/TTOT setting always raises inspiratory flow. The timing effects of increasing fM at a fixed inspiratory-flow setting cannot be predicted without knowledge of the patient’s actual trigger rate.
τ | Time constant |
AC | Assist-control mode |
ARDS | Adult respiratory distress syndrome |
CMV | Controlled mechanical ventilation |
CPAP | Continuous positive airway pressure |
Ers | Elastance of the respiratory system |
Edi | Electromyographic tracing of the diaphragm |
F | Force |
fA | Actual breathing rate |
FEF25–75 | Forced expiratory flow in the mid vital capacity range |
FIO2 | Fractional inspired oxygen concentration |
fM | Machine backup rate |
I:E ratio | Inspiratory-to-expiratory time ratio |
IMV | Intermittent mandatory ventilation |
PaCO2 | Arterial CO2 tension |
PaO2 | Arterial O2 tension |
Paw | Airway pressure |
PCV | Pressure-controlled ventilation |
PEEP | Positive end-expiratory pressure |
PEEPE | Extrinsic positive end-expiratory pressure |
PEEPi | Intrinsic positive end-expiratory pressure |
Pel | Elastic recoil pressure |
Ptp | Transpulmonary pressure |
Pmus | Inflation pressure exerted by inspiratory muscles |
Pres | Resistive pressure |
Prs | Recoil of respiratory system |
PSV | Pressure support ventilation |
SIMV | Synchronized intermittent mandatory ventilation |
TE | Expiratory time |
TI | Inspiratory time |
TI/TTOT | Duty cycle |
TTOT | Total cycle time |
TLC | Total lung capacity |
![]() | Flow |
![]() | Ventilation-perfusion ratio |
![]() | Volume of CO2 produced in liters per minute |
![]() | Minute ventilation |
![]() | Mean inspiratory flow |
V(t) | Instantaneous lung volume |
![]() | Dead-space-to-tidal-volume ratio |
Vee | Volume of lungs at end expiration |
Vrel | Relaxation volume |
VT/TE | Mean expiratory flow |
VT/TI | Mean inspiratory flow |
VT | Tidal volume |
Vtrapped | Volume of gas remaining in the elastic element at the beginning of a new machine inflation |
Wel | Elastic work |
During pressure-preset ventilation, the ventilator applies a predefined target pressure to the endotracheal tube during inspiration. The resulting VT and inspiratory flow profile varies with the impedance of the respiratory system and with the strength and duration of the patient’s inspiratory efforts. Therefore, when the lungs or chest wall become stiff, airway resistance increases, the patient’s own inspiratory efforts decline, or TI decreases, VT decreases. An increase in respiratory system impedance can lead to a dangerous fall in minute ventilation (), hypoxemia, and CO2 retention, but in contrast to volume-preset modes, it does not predispose the patient to an increased risk of barotrauma. On the other hand, pressure-preset modes are no safeguard against ventilator-induced lung injury because large fluctuations in respiratory impedance or patient effort would result in large VT fluctuations directly undermining the primary objective of lung-protective mechanical ventilation (see section on Acute Lung Injury and Hypoxic Respiratory Failure).
Pressure-support ventilation (PSV), pressure-controlled ventilation (PCV) and airway pressure release ventilation (APRV) are the most widely used forms of pressure-preset ventilation. In contrast to PCV, PSV requires the patient’s effort before a machine breath is delivered. Consequently, PSV is not suitable for the management of patients with central apneas. During PCV, the physician sets the machine rate, the TI, and thus the I:E ratio. In PSV, phase switching is linked to inspiratory flow, which, in turn, depends on the impedance of the respiratory system, as well as on the timing and magnitude of inspiratory muscle pressure output.11,14 APRV is akin to PCV with a long duty cycle, but with one important distinction: patients are able to take spontaneous breaths throughout all phases of the machine cycle.
PSV remains a popular weaning mode for adults. Its popularity is based on the premise that weaning from mechanical ventilation should be a gradual process and that the work of unassisted breathing through an endotracheal tube is unreasonably high and could lead to respiratory muscle failure in susceptible patients. Actual measurements of pulmonary resistance and work of breathing before and after extubation do not support this reasoning,17,18 and several large clinical trials have established equivalence between PSV and T-piece weaning (unassisted breathing from a bias-flow circuit).19–21 In the PSV mode, a target pressure is applied to the endotracheal tube, which augments the inflation pressure exerted by the inspiratory muscles (Pmus) on the respiratory system. When inspiratory muscles cease to contract and Pmus falls, inspiratory flow (a ventilator-sensed variable) declines, and the machine switches to expiration. Early PSV modules were designed to generate pressure ramps (square wave inflation pressure) and had relatively rigid flow-based, off-switch criteria. Most recent versions of PSV afford control over the rate of rise in inspiratory pressure and the flow threshold at which inspiration is terminated.11,14,22
During synchronized intermittent mandatory ventilation, a specified number of usually volume-preset breaths are delivered every minute. In addition, the patient is free to breathe spontaneously between machine breaths from a reservoir or to take breaths augmented with PSV. Most ventilators allow the operator to choose between volume- and pressure-preset mandatory breaths. Unless the patient fails to breathe spontaneously, machine breaths are delivered only after the ventilator has recognized the patient’s effort; that is, ventilator and respiratory muscle activities are “synchronized.” Because nowadays all IMV circuits are synchronized, the terms IMV and synchronized intermittent mandatory ventilation are used interchangeably. Although synchronized intermittent mandatory ventilation remains a viable and popular mode of mechanical ventilation, compared with the alternatives, PSV and T piece, it has clearly proven inferior as a weaning modality.19,20,23,24 Moreover, the care provider needs to be aware of certain pitfalls when using IMV. Even a small number of volume-preset IMV breaths per minute may make the blood-gas tensions look acceptable in patients who otherwise meet criteria for respiratory failure. One should suspect this in patients with small spontaneous VT (≤3 mL/kg of body weight), in those with thirty or more inspiratory efforts per minute regardless of whether they trigger a machine breath, and when dyspnea and thoracoabdominal paradox indicate a heightened respiratory effort. One reason that IMV remains popular is because it silences apnea alarms by masking PSV-induced respiratory dysrhythmias, which are common in sleeping and obtunded patients.25–27
Many new-generation mechanical ventilators feature modes with closed-loop feedback control of both pressure and volume.28,29 While a detailed description of the operating principles of every new mode is beyond the scope of this chapter, it is important to understand the rationale behind dual-control modes and some of their general operating characteristics. The idea behind most dual-control modes is the meeting of a ventilation target while maintaining low inflation pressures. To this end, ventilator output is adjusted based on volume, flow, and pressure feedback. This may occur within each machine cycle or gradually from one cycle to the next. Modes that adjust output within each cycle execute a predetermined pressure-time program as long as the desired VT is reached. When the VT target is not reached, inspiration continues at a preselected inspiratory flow rate (volume-limited) until the target volume is attained. Volume-assured pressure support and pressure augmentation are examples of such modes.30 Breath-to-breath dual-control modes are pressure-limited and time-cycled or flow-cycled. Ventilator output is derived from the pressure–volume relationship of the preceding breath and is adjusted within predefined pressure limits to maintain the target VT. Adaptive support ventilation, pressure-regulated volume control, volume control+, autoflow, adaptive pressure ventilation, volume support, and variable pressure support are examples of breath-to-breath control modes. There is no evidence that the use of dual-control modes improves patient outcomes.31,32 Moreover, there is a conceptual problem insofar as less complex modes already safeguard against hypoventilation, whereas dual-control modes do little to protect the patient from a potentially harmful increase in the regulated variable, that is, large VT-mediated lung injury.33,34
Neurally adjusted ventilatory assistance (NAVA) and proportional-assist ventilation (PAV)35–37 are the most complex, and arguably the most promising, closed-loop ventilation modes. During NAVA, the diaphragm’s electrical activity is recorded with an esophageal probe, and the signal is conditioned and transposed into a positive airway pressure output. During PAV, the ventilator derives its mechanical output from continuously monitored Paw, V, and information, which, in turn, reflects Pmus. The operating principles of PAV will be easier to understand after a review of patient–ventilator interactions (see “The Mechanical Determinants of Patient-Ventilator Interactions” below). Compared to conventional modes of mechanical ventilation, both NAVA and PAV preserve the biologic variability in breathing rate and VT, which is generally considered lung protective.38 Moreover, the maintenance of intrinsic respiratory control mechanisms is likely to reduce the probability of exposing the lungs to injurious deformations. Although there is ample literature on the effects of closed-loop modes on patient–ventilator interactions and physiologic end points, there is insufficient clinical experience to judge efficacy of these modes compared to conventional approaches. This is particularly true for NAVA, which was only recently introduced to the world market, but holds particular promise as platform for delivering noninvasive mechanical ventilation and as a support mode for neonates and small infants. Be this as it may, the full-scale migration of closed-loop modes from expert hands into general practice will likely depend on the willingness of providers to acquire the physiologic insights and skills necessary for managing patients who are ventilated with “unconventional” modes.
The Mechanical Determinants of Patient–Ventilator Interactions
It is useful to think of patient–ventilator interactions in terms of a mechanical or electrical analog system consisting of a resistive element (resistor) and an elastic element (capacitor) in series. The forcing function is defined by the pressure or flow “program” that is executed by the mechanical ventilator.
In Figure 5-2, a piston pump (the mechanical ventilator) is attached to a rigid tube (the resistive element) and a balloon (the elastic element). An in-series mechanical arrangement means that at any time t, the pressure that is applied to the tube inlet Pi(t) (near the attachment to the ventilator) is equal to the sum of two pressures, an elastic pressure Pel(t) and a resistive pressure Pres(t):
Figure 5-2
Components of inlet pressure. The model of the respiratory system at right consists of a resistive element (straight tube) and an elastic element (balloon) connected to a ventilator (piston). During inflation of the model with constant flow (lower panel), there is a stepwise increase inlet pressure (Pi) that equals the loss of pressure across the resistive element (Pres) (upper panel). Thereafter, Pi increases linearly and reflects the mechanical properties of the elastic element (Pel). Pi is the sum of Pres and Pel. At end inspiration, when flow has ceased (Insp. Pause), Pi decreases by an amount equal to Pres; Pi equals Pel during Insp. Pause. TI, inspiratory time; TE, expiratory time. (Used, with permission, from Hubmayr, et al. Physiologic approach to mechanical ventilation. Crit Care Med. 1990;18:103–113.)
The tube outlet pressure at the junction with the balloon is equal to the pressure inside the balloon, that is, Pel. Pres is the difference in pressure between the tube inlet and the tube outlet. Assuming linear-system behavior, the inlet pressure-time profile can be computed for any piston stroke volume (Vstroke) and flow () setting, provided the resistive properties of the tube (R) and the elastic properties of the balloon (E) are known:
The elastance (E) is a measure of balloon stiffness and is equal to the Pel-to-V stroke ratio (assuming 0 volume and pressure at the beginning of balloon inflation). Therefore, Pel(t) in Eq. (1) can be replaced with EV(t) in Eq. (2). Because the Ohm law states that the tube resistance (R) is equal to the Pres-to- ratio, Pres(t) in Eq. (1) can be replaced with the product
in Eq. (2).
Equations (1) and (2) are based on the equation of motion, which describes the force (F) that must be applied to a mass (M) in order to move it a certain distance (d) at a rate dd/dt against a spring (elastic) load:
where k = stiffness of the spring (analogous to E); k′ = frictional resistance between mass and supporting surface (analogous to R); and k″ = inertance, which is proportional to mass.
The first and second derivatives of d (analogous to volume) represent the velocity (dd/dt, analogous to flow) and the acceleration [d(dd/dt)/dt] of the mass at time t, respectively. As long as the mass of the moving parts in the model of Figure 5-2 is small, any inertive-pressure component that is dissipated during the acceleration of gas at the beginning of the pump instroke can be ignored. Therefore, the respiratory analog of the equation of motion [Eqs. (1) and (2)] considers only elastic and resistive pressures.
Consider the Pi-time profile of a tube-balloon system with resistance of 10 cm H2O × L/s and an elastance of 10 cm H2O when the piston pump is programmed to deliver a volume of 0.5 L with a constant (square wave) flow of 0.5 L/s. Because flow and R remain constant throughout inflation, Pres is constant at 5 cm H2O and accounts for the initial step change in inlet pressure at the beginning of inflation. As gas enters the balloon, Pi increases further and reaches a value of 10 cm H2O at end inflation. At that instant, the tube is occluded (end-inflation hold), causing Pi to drop by an amount equal to Pres (as flow returns to 0). The end-inflation hold pressure is equal to Pel at that volume. Its value of 5 cm H2O is equal to the product of piston stroke volume (0.5 L) and elastance (10 cm H2O/L), as follows from Eqs. (1) and (2). Subtracting Pres from Pi(t) yields the Pel per time course during inflation. Pel increases linearly with time and volume. Its rate of rise (dPel/dt) parallels that of Pi and is determined by E and the flow setting of the piston:39
Although changes in inspiratory flow result in proportional changes in dPel/dt, flow has no effect on peak Pel, provided that Vstroke, and thus peak lung volume, is held constant. This is in contrast to peak Pi, which reflects flow-dependent changes in Pres, as well as change in Pel, at end inflation. The relevance of this important property of linear single-compartment systems will become apparent later when the relationships between ventilator settings and barotrauma (balloon yield stress) are discussed (see “Acute Lung Injury and Hypoxic Respiratory Failure” below).
In mechanically ventilated subjects, expiration is usually a passive process that is driven by the elastic recoil (Pel) of the respiratory system. Assuming linear pressure-volume and pressure-flow relationships, the instantaneous expiratory flow [] is given by
Because Pel(t) is a function of E and of the instantaneous lung volume [V(t)], Eq. (5) can be rewritten as
where C (the compliance of the respiratory system) is simply the inverse of the elastance (E). The product of R and C characterizes the time constant (τ) of single-compartment linear systems. The time constant defines the time at which approximately two-thirds of the volume above Vrel has emptied passively. From this it should be clear that patients with increased respiratory system resistances and compliances (e.g., patients with emphysema) are prone to dynamic hyperinflation even if one ignores nonlinear system behavior, such as flow limitation, for the moment.
The volume of gas remaining in the elastic element at the beginning of a new machine inflation (Vtrapped) can be calculated as follows:
In other words, the degree of dynamic hyperinflation is determined by the choice of ventilator settings, specifically mean expiratory flow (VT/VE) and the time constant of the respiratory system, which reflects its mechanical constants R and C.40 These important concepts are expanded on under “Obstructive Lung Diseases” below.
Before linear model principles are applied to the ventilator management of patients, one must be cognizant of the model’s limitations. The limitations fall into two general categories: those related to nonlinear respiratory system characteristics and those related to respiratory muscle activation during mechanical ventilation. Sources of nonlinear system behavior include inhomogeneities within the numerous bronchoalveolar compartments (particularly when the lungs are diseased),41 respiratory system hysteresis from recruitment of alveolar units and time-dependent surface tension phenomena,42 and phenomena related to dynamic airway collapse and expiratory flow limitation.43 Coactivation of the respiratory muscles during mechanical ventilation invalidates Eqs. (1) through (7) insofar as they alter the impedance of the respiratory system and change the driving pressure for expiratory flow. If one assumes that the respiratory muscles and the ventilator are arranged in series, then the monitoring of pressure, volume, and flow at the airway opening offers the opportunity to define the magnitude, rate, and duration of respiratory muscle output in mechanically ventilated subjects.44,45
Defining Therapeutic End Points in Common Respiratory Failure Syndromes
Numerous diseases of cardiopulmonary systems can cause respiratory failure. From a ventilator management perspective, it is useful to group them into those that cause lung failure and those that cause ventilatory pump failure.
The hallmark of lung failure is hypoxemia, which is usually the result of severe ventilation-perfusion mismatch. The hallmark of ventilatory pump failure is hypercapnia. Ventilatory pump failure may be caused by disorders of the central nervous system, peripheral nerves, or respiratory muscles. It also may accompany diseases of the lungs, such as emphysema, once the ventilatory pump fails to compensate for inefficiencies in pulmonary CO2 elimination. Two classic examples of hypoxic and hypercapnic ventilatory failure that require fundamentally different approaches to mechanical ventilation are the acute respiratory distress syndrome (ARDS) and chronic airflow obstruction. The therapeutic goal in ARDS is to protect the lung from mechanical injury while raising lung volume in an attempt to reduce shunt by reexpanding collapsed and flooded alveoli. In contrast, the therapeutic goal in a patient with hypercapnic ventilatory failure from exacerbation of airways obstruction is to reduce dynamic hyperinflation and to protect the respiratory muscles from overuse.
Acute Lung Injury and Hypoxic Respiratory Failure
Acute lung injury (ALI) is a syndrome associated with bilateral pulmonary infiltrates and a gas-exchange impairment severe enough to lower the arterial oxygen tension-to-fractional inspired oxygen concentration ratio (PaO2/FIO2) below 300.46 Heart failure and moderate to severe preexisting chronic lung disease must be absent. ALI and its more severe form, ARDS, are often complications of systemic illnesses such as sepsis.47 The impairment on pulmonary gas exchange therefore is accompanied frequently by microcirculatory failure. The general management goal in these disorders is to augment systemic oxygen delivery until the metabolic demands of the organism can be met. This goal requires an integrated approach between cardiovascular and ventilator support.48
Ventilator support is often difficult because exceedingly high ventilatory requirements challenge the performance capacity of mechanical ventilators; render patients at risk for barotrauma, ventilator-induced lung injury, and cardiovascular collapse; and often are accompanied by excessive respiratory muscle activity (“fighting the ventilator”). All these conditions on occasion can necessitate heavy sedation and neuromuscular blockade.
The two principal means by which the physician can increase PaO2 in ARDS are to raise the FIO2 and to elevate the volume about which the lungs are being ventilated. The danger inherent in raising FIO2 is oxygen toxicity,49 whereas manipulating lung and/or VT may result in ventilator-induced lung injury34,50 and/or barotrauma.51 Presented with the choice between two different kinds of adverse reactions, physicians currently tend to be more fearful of mechanical lung injury than of oxygen toxicity. Unfortunately, there are no clinical outcome studies that shed light on the interactions between these two iatrogenic insults. It is common practice to initiate ventilator support with an FIO2 of 1.0 and to ignore the potential for oxygen toxicity during the first few hours of ventilator management. Although, generally speaking, the relative and combined risks of oxygen toxicity and overdistension injury of the lungs remain poorly defined, there are instances in which it seems wise to minimize FIO2, namely, in patients who have received drugs such as bleomycin or amiodarone, which make the lungs particularly susceptible to reactive O2 species-mediated injury.52
The insults to the lungs of patients with hypoxic respiratory failure are often patchy and result in flooding and closure of dependent airspaces (Fig. 5-3).53,54 Paraspinal regions of the lung appear most susceptible to atelectasis (lack of aeration) because, in the supine posture, they normally empty close to their residual volume and they receive most of the pulmonary blood flow.55 Therefore, insults to their capillary integrity are most likely to promote alveolar flooding, closure of airspaces by liquid plugs, surfactant inactivation, and gas-absorption atelectasis.56 The accumulation of airway liquid and foam also generates interfacial forces that are large enough to abrade the epithelial lining of small airways during breathing, causing further injury.57–61 Ventilator management therefore must be directed toward preventing the repeated opening and closing of unstable lung units, which means reestablishing aeration and ventilation of the flooded lung (Fig. 5-4).62
Figure 5-3
Computed tomographic (CT) scan of a patient with acute respiratory failure in the supine position. Note the patchy, nonuniform distribution of alveolar edema. (Used, with permission, from Gattinoni L, et al. Body position changes redistribute lung computed-tomographic density in patients with acute respiratory failure. Anesthesiology. 1991;74:15–23.)
Figure 5-4
Schematic of the therapeutic end points of ventilator management in ARDS. Raising peak lung volume toward total lung capacity (TLC) increases the risk of barotrauma. Keeping the minimal volume near relaxation volume (Vrel) raises the likelihood of alveolar derecruitment at end expiration and the need to apply large opening pressures so as to recruit collapsed and flooded lung regions during the subsequent breath.
There are several ways to achieve this objective: (a) by raising overall lung volume through the judicious use of extrinsic positive end-expiratory pressure (PEEP), (b) by raising lung volume dynamically through “intentional gas trapping,” (c) by increasing VT, and (d) by taking advantage of the local distending forces generated by an actively contracting diaphragm. Any one of these approaches may be combined with so-called recruitment maneuvers, which consist of sustained (up to 40 seconds) inflations of the lungs to high volumes and pressures.63–65 The preferred and time-tested approach is the judicious use of extrinsic PEEP. All the other means of raising lung volume are comparatively untested, and in the case of VT, manipulations can be outright harmful.33,34 Although there is a strong physiologic rationale to condition (i.e., “open”) the lungs with recruitment maneuvers before a PEEP adjustment, most experimental and clinical data indicate that conditioning effects are relatively short-lived.66–69 Because it is common for patients with ALI to have an increased respiratory rate, a component of dynamic hyperinflation is often present.70 Despite the short time constant for lung emptying, the use of extrinsic PEEP valves, which in older-generation ventilators represent resistive as well as threshold loads, and ventilator settings that require large mean expiratory flows (VT/TE; see “Mean Expiratory Flow: The Hidden Variable” below) contribute to dynamic hyperinflation.
Although the experimental evidence in support of PEEP therapy in injured lungs is overwhelming, its specific application to clinical practice remains controversial. There is general agreement among experts that patients with injured lungs should be ventilated with PEEP settings greater than 5 cm H2O. The risk, however, of overinflating and thereby damaging well-aerated, generally nondependent lung units and adverse hemodynamic effects set limits to an aggressive recruitment strategy.71,72 Uncertainty about the topographic distribution of lung parenchymal stress and related stress injury thresholds are partly the reason why there is no consensus as to whether PEEP should be set arbitrarily to 10, 15, or 20 cm H2O, whether it should be targeted to specific physiologic end points, and, if so, what those end points and their specific target thresholds should be. Several large randomized clinical trials have failed to resolve the controversy about “best PEEP.”73–75 Although none of these trials established superiority of one specific PEEP strategy over another, proponents of aggressive lung recruitment argue that PEEP was not targeted to the appropriate surrogate end points. Specifically, lung recruitment, chest wall recoil, and parenchymal stress were not measured or considered in the choice of PEEP settings.
Tools for assessing recruitment responses include (a) measures of regional lung aeration with computed tomography or electrical impedance imaging of the chest,76–78 (b) measurement of lung and/or respiratory system pressure–volume relationships,79–82 and (c) assessment of within-breath oscillations in arterial O2 tension with indwelling arterial O2 sensors with fast response times.83,84 At the bedside, the most readily available PEEP management guides are airway inflation pressure amplitude, ΔP (in case of volume preset ventilation) or VT (in case of pressure preset ventilation). As long as raising PEEP causes recruitment of previously “closed” lung units without overdistending already open ones, ΔP will decrease, reflecting the corresponding increase in compliance. In relaxed patients who are being ventilated with a pressure preset mode, the PEEP-related effect on lung compliance can be inferred from corresponding VT changes. Adjusting PEEP until ΔP reaches a minimum, or conversely in the case of pressure preset ventilation until VT
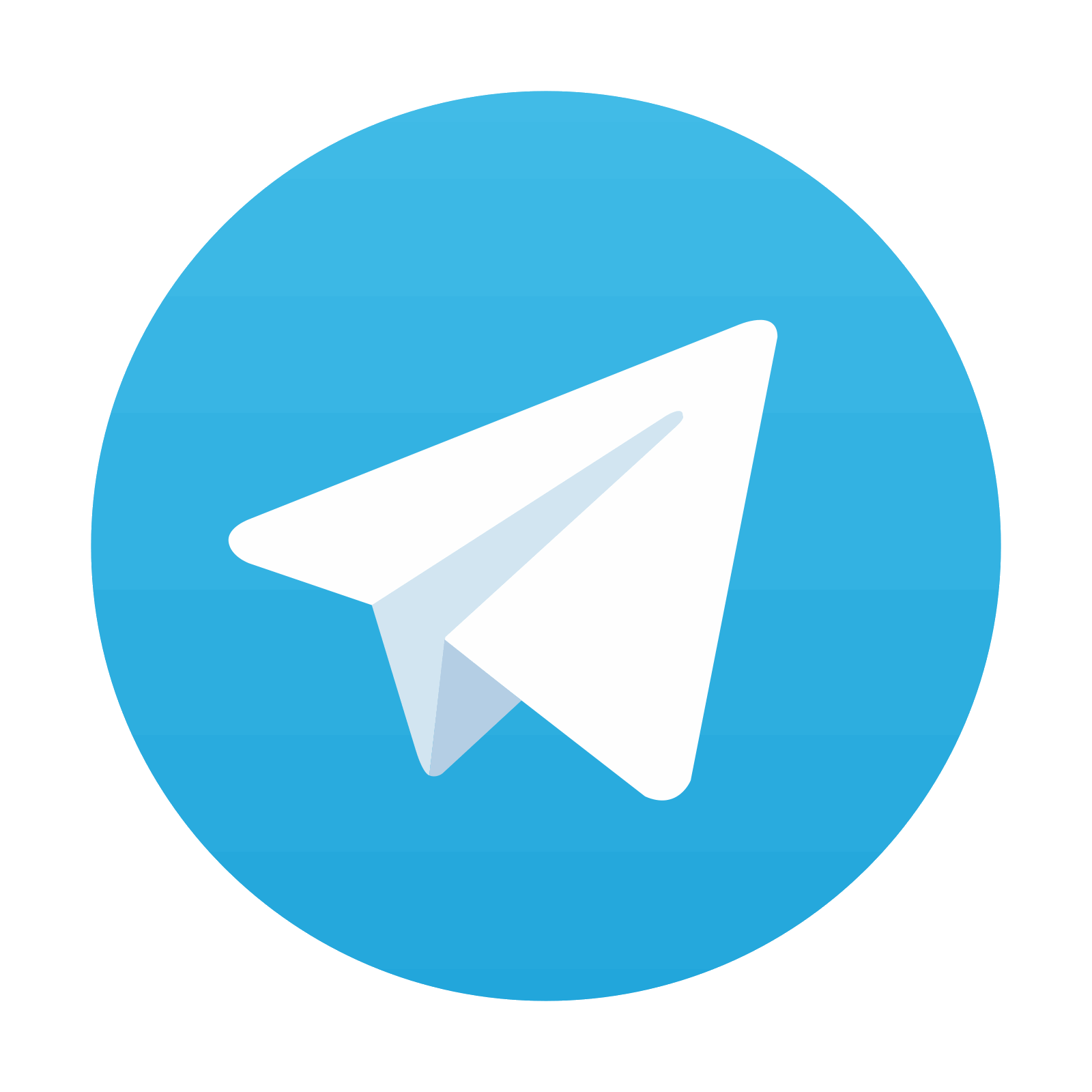
Stay updated, free articles. Join our Telegram channel
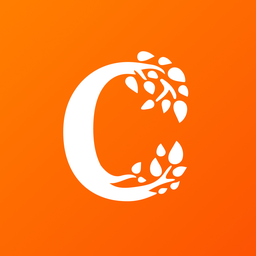
Full access? Get Clinical Tree
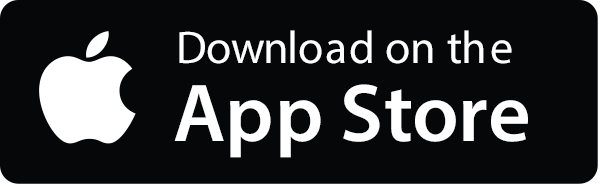
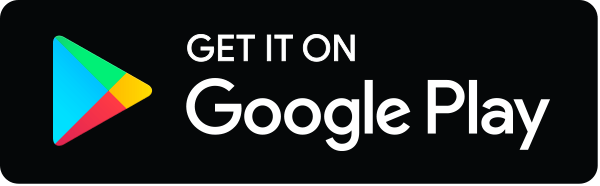
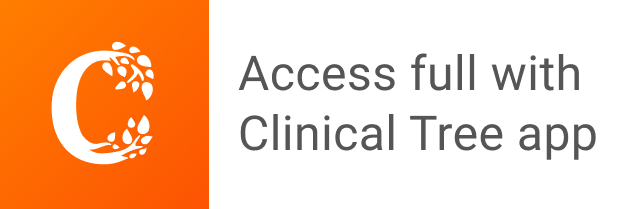