Chapter 103 Sepsis
Epidemiology
A true picture of the epidemiology of septic shock is clouded by the lack of a reliable case definition. This is true for both the adult and pediatric populations. A few pediatric-specific studies, however, illustrate the importance of septic shock in today’s modern intensive care unit (ICU). Proulx et al. analyzed the incidence and outcome of the systemic inflammatory response syndrome (SIRS), sepsis, severe sepsis, and septic shock (see next section for definitions) in a single institution.1 A total of 1058 admissions were analyzed over a 1-year period. SIRS was present in 82% of patients, 23% had sepsis, 4% had severe sepsis, and 2% had septic shock. The overall mortality rate for this patient population was 6%, with the majority of deaths occurring in patients with multiple organ dysfunction syndrome (MODS). Among the patients with MODS, distinct mortality rates were associated with SIRS (40%), sepsis (22%), severe sepsis (25%), and septic shock (52%). Later studies by Watson et al. provide the most comprehensive epidemiologic surveys of pediatric septic shock to date.2 By linking 1995 hospital records from seven large states (representing 24% of the United States population) with census data, they estimated an incidence of 42,371 cases of severe sepsis in individuals younger than 20 years of age (0.6 cases/1000 population). The highest incidence was in neonates (5.2 cases/1000 population), compared with children ages 5 to 14 years who had an incidence of 0.2 cases/1000 population. The overall mortality rate in this population was 10.3% (4364 deaths per year nationally). In addition, patients younger than 1 year of age and patients with comorbidities had higher mortality rates than patients between 5 and 14 years old and patients without comorbidities, respectively. Their study also estimated an annual national health care cost of $1.7 billion associated with severe sepsis.
In a follow-up study, Watson et al.3 compared the epidemiology of severe sepsis between 1995 and 1999. Using a similar strategy as described for their earlier study, they found a 13% increase in the absolute number of cases of severe sepsis during this time period. The majority of the increase was accounted for by severe sepsis in children younger than 1 year of age. In contrast, the overall mortality rate for severe sepsis decreased from 10.3% to 9.0% during this time period.
In the most recent report on the epidemiology of pediatric sepsis, Czaja and colleagues used the discharge diagnosis of severe sepsis for Washington State to investigate the readmission rates and late mortality for children (1 month to 18 years old) following severe sepsis.4 From 1990 through 2004, 7183 children were diagnosed with severe sepsis and 6.8% of these patients died during the sentinel admission or within 28 days of discharge. Importantly, death certificates confirmed that an additional 434 (6.5%) of the initial survivors died during the follow-up period with the highest late death rate occurring within 2 years of the initial hospitalization. Although most of the early, as well as the late deaths occurred in children with comorbidities (8% early death, 10.4% late death), 8% of children with no comorbidities died during their initial hospitalization with 2% of the 28-day survivors being classified as late deaths.
Collectively, these data illustrate that septic shock continues to present a major public health problem in terms of incidence, mortality, and health care costs. Nevertheless, there is an ongoing need for quality epidemiologic studies of septic shock in children. Quality epidemiologic studies are necessary for our understanding not only of incidence, but also of the impact of new knowledge and therapies. One major issue that must be addressed is the development of more meaningful and consistent case definitions. Consistent case definitions will also facilitate and improve the design of more effective interventional trials specific to the pediatric population. Equally important is objectively measuring long-term outcomes in these patients (i.e., quality of life) beyond the dichotomy of “alive” or “dead.” Progress in this important area is steadily coming to fruition.5–8
Definitions
The International Consensus Conference on Pediatric Sepsis and Organ Dysfunction was convened in 2002 to develop pediatric-specific definitions for SIRS, sepsis, severe sepsis, septic shock, and organ failure, and the results of this conference were subsequently published.9 The standard terms to describe the sepsis spectrum are systemic inflammatory response syndrome (SIRS), sepsis, severe sepsis, and septic shock. Each term is intended to describe a clinical syndrome having increasing illness severity and relatively increasing specificity, which in turn drives important clinical decision and therapeutic processes.
SIRS is not a diagnosis. The term is intended to represent a state of relative inflammatory/immune activation in a given patient, and is said to be present when a patient meets at least two of the four criteria listed in Box 103-1 one of which must be abnormal temperature or abnormal leukocyte count. Thus, patients with diverse clinical conditions, such as sepsis, pancreatitis, burns, or hypermetabolism following major trauma or surgery, can meet criteria for SIRS. Sepsis is defined as SIRS secondary to an infection, either documented by microbiology cultures or in the presence of other clinical evidence of infection. Severe sepsis is defined by sepsis criteria plus either cardiovascular dysfunction or acute respiratory distress syndrome, or at least two other dysfunctional organ systems. Septic shock is defined by sepsis criteria, plus cardiovascular dysfunction. Importantly, each criterion takes into account the influence of developmental age on physiological variables. The reader is referred to the original publication by Goldstein et al.9 for further details and definitions of organ dysfunction.
Box 103–1 Criteria for SIRS
Clinical Presentation
As a syndrome that potentially affects the entire body, the clinical presentation of sepsis is highly heterogeneous. The most common clinical manifestations of sepsis include fever or hypothermia, tachypnea, tachycardia, leukocytosis or leukopenia, thrombocytopenia, and change in mental status. It should be noted however that, in the absence of meningitis, changes in mental status are relatively late manifestations of septic shock and should not be relied upon for early recognition of shock. One of the earliest signs that alerts caregivers to the possibility of infection is fever. A number of the cytokines elicited in response to infection are pyrogens, particularly interleukin (IL)-1β and tumor necrosis factor (TNF)-α. Patients can also have hypothermia, which is more common in infants than older children. Finally, petechiae and/or purpura can be present and are potentially ominous signs of purpura fulminans.10
The degree to which an individual patient manifests these physiologic perturbations is highly variable. In some cases, patients display increased cardiac output with diminished systemic vascular resistance. The presenting symptoms in this type of patient are tachycardia, a hyperdynamic precordium, bounding pulses, and warm, flushed skin characteristic of the distributive mode of shock or the so-called “warm” shock state. Despite this clinical appearance, the perfusion of major organs during warm shock may remain highly compromised secondary to maldistribution of blood flow. Alternatively, a patient with depressed cardiac output and elevated systemic vascular resistance has cool, mottled skin with diminished pulses and poor capillary refill characteristic of the “cold” shock state. Limited data and our collective anecdotal experience suggest that this latter presentation, cold shock, is more common in younger children compared to teenagers and adults.11 Recently, it has been suggested that patients who develop community-acquired septic shock more commonly present to the ICU with signs of “cold” shock; whereas patients that develop septic shock secondary to catheter-related infections more commonly present to the ICU with signs of “warm” shock.12 It is important to recognize that a given patient may transition from one shock state to another, and that recognition and reassessment of these classes of shock are absolutely central to the choice of cardiovascular medications.
All organ systems can be adversely affected by poor perfusion and decreased oxygen delivery. In addition, all organ systems can be directly or indirectly injured by bacterial toxins, circulating cytokines, and the products of activated white blood cells. The end result of these complex and interrelated pathologic mechanisms is multiple organ dysfunction syndrome, which describes the serial and progressive failure of various organ systems, and is associated with increased morbidity and mortality.13–15
Pathogenesis
A large number of clinical and basic science studies have focused on the mechanisms underlying the development of sepsis. At least three major hypotheses have been proposed to explain the development of sepsis and its sequelae. The first hypothesis attributes the development of sepsis to an excessive or uncontrolled host inflammatory response. This “proinflammatory” hypothesis is broadly consistent with the concept of SIRS, and is generally well supported by experimental and clinical data. However, a large number of clinical trials aimed directly at inhibition of various components of this putative excessive inflammatory response have failed, thus leading to the development of alternative hypotheses. One such alternative hypothesis states that sepsis is not directly the result of excessive inflammation, but rather a more direct manifestation of failed antiinflammatory responses. Thus in this alternative hypothesis there is direct failure of the compensatory antiinflammatory response syndrome (CARS), which subsequently permits unchecked proinflammatory responses. Related to the CARS concept is the concept of immunoparalysis, which embodies the third overall hypothesis to account for the clinical manifestations of sepsis. The hypothesis of immunoparalysis postulates that sepsis is not a manifestation of too much or too little inflammation, but rather a form of acquired immunodeficiency (both innate and adaptive immunity), leading to an inability to effectively clear pathogens and their products, which thereby cause direct tissue and organ injury.16,17
A conceptual framework for integrating these three hypotheses/paradigms is provided in Figure 103-1. All three paradigms are biologically plausible and supported by the existing literature.18 While seemingly vastly different in concept, they are not mutually exclusive in the context of a highly heterogeneous syndrome such as human sepsis. That is, it is plausible that all three paradigms are valid across a given cohort of heterogeneous patients with sepsis. In addition, each paradigm has the potential to influence all of the other paradigms, as indicated in Figure 103-1. The following sections review the existing literature supporting these three paradigms and will serve to frame the important concept of heterogeneity in sepsis. A major challenge in the field of sepsis is to more effectively understand how a given patient fits into one of these three paradigms (i.e., stratify or phenotype).
Pathogen Recognition and Signal Transduction
The fundamental role of the immune system is to detect, contain, and eradicate invading pathogens. The first step in this process involves pathogen recognition, which is achieved by the activation of pattern recognition receptors (PRRs) on immune cells by pathogen-associated molecular patterns (PAMPs).19 Examples of PAMPs include lipopolysaccharide from the cell walls of gram-negative bacteria; lipoteichoic acid from the cell walls of gram-positive bacteria; mannans from the cell walls of yeast; double-stranded RNA of viruses; and unmethylated, CpG-rich DNA unique to bacterial genomes. The most well studied PRRs include the family of toll-like receptors (TLRs), which can have relatively specific recognition of PAMPS.19 For example, TLR-4 recognizes lipopolysaccharide, wherease TLR-2 recognizes lipoteichoic acid. Other examples of PRRs or PRR components include CD-14, scavenger receptors, NOD receptors, pentraxins, and collectins.20
Much of the activation of the immune system upon PRR activation relies upon signal transduction mechanisms, which serve to transfer the signal of pathogen recognition at the cell surface to the intracellular compartment in order to induce new gene expression or a change in cellular function. One of the major signal transduction mechanisms of the immune system is the NF-κB pathway, which serves as a master “switch” for the expression of a wide variety of genes involved in inflammation and immunity. Indeed, activation of the NF-κB pathway is a major signaling pathway in the pathophsiology of sepsis, and may represent a potential therapeutic target.21,22 Another major signaling pathway for the regulation of genes involved in inflammation and immunity is the mitogen-activated protein kinase (MAPK) signaling pathway. The MAPKs consist of three major families: p38 MAPK, extracellular-regulated protein kinase (ERPK), and c-Jun N-terminal kinases (JNK). These major kinase families are also referred to as a stress-activated protein kinases (SAPKs). Similar to the NF-κB pathway, the MAPKs are also regarded as potential therapeutic targets in the context of sepsis.21,23,24 Finally, there is now increased attention on the phosphatase family of intracellular signaling molecules in the context of sepsis. Whereas kinases direct cellular signaling by adding phosphate groups to intracellular signaling proteins, phosphatases serve to remove phosphate groups from these same intracellular signaling proteins and can thus serve to modulate proinflammatory cell signaling.25,26
Cytokines as Principal Mediators of the Sepsis Response
Cytokines represent a broad family of proteins that have paracrine, autocrine, and endocrine properties, and the ability to regulate and modulate virtually all aspects of immunity and inflammation. Common features of cytokines are provided in Box 103-2. Herein we will review a selected group of cytokines thought to play an important role in the pathophysiology of sepsis.
Tumor Necrosis Factor-α
Tumor necrosis factor alpha (TNF-α) is perhaps the most well-studied cytokine that is causally linked to sepsis. Evidence for TNF-α mediation of sepsis includes the observations that TNF is produced by hematopoietic cells; its expression is temporally related to the development of shock; it can by itself induce experimental septic shock in animals; and passive immunization against TNF blunts the endotoxin-induced sepsis response.27 The proinflammatory effects of TNF include leukocyte-endothelial cell adhesion, transformation to a procoagulant phenotype, induction of inducible nitric oxide synthase, and functioning as a principal “early” cytokine that induces the subsequent cascade of mediators and cytokines promulgating the septic response. Despite a plethora of preclinical studies demonstrating the important proximal role of TNF-α in the pathophysiology of sepsis, multiple clinical trials targeted at neutralization of TNF-α activity have thus far failed to demonstrate efficacy.28
Interleukin-1β
Interleukin (IL)-1β has many redundant biological properties to those of TNF-α, and is also considered to be a major early cytokine in the sepsis response.29 IL-1β leads to inflammatory and immune cell activation via the NF-κB and MAPK pathways. Also, similar to TNF-α, clinical trials targeted at neutralization of IL-1β activity have thus far failed to demonstrate efficacy despite promising preclinical data.
Interleukin-6
Interleukin-6 expression is highly dependent on TNF-α and IL-1β, and is consistently found to be elevated during the course of sepsis.30 IL-6 is a pleiotropic cytokine possessing a number of functions including driving the acute-phase response in hepatocytes, differentiating myeloid cells, stimulating immunoglobulin production, and activating T-cell proliferation.31 Because increased IL-6 admission levels have been correlated with death in the context of sepsis, there has been interest in using IL-6 as a stratification biomarker for interventional clinical trials in sepsis. While this stratification approach has been highly effective in animal models of sepsis,32 it has thus far failed when applied in the clinical setting.33
Interleukin-8
Interleukin-8 is a canonical member of the chemokine subclass of cytokines.34 The term “chemokine” refers to the ability of certain cytokines to serve as chemoattractants, which direct leukocyte movement to sites of infection and inflammation (chemotaxis). Both TNF-α and IL-1β can induce IL-8 production from a variety of cells, including endothelial cells, macrophages, neutrophils, and epithelial cells. IL-8 is the principal human chemoattractant for neutrophils, and appears to play a major role in the recruitment of neutrophils to the lungs in patients with sepsis-induced acute respiratory distress syndrome.35 Recently, it was demonstrated that serum IL-8 measurements within 24 hours of presentation to the ICU can robustly predict good outcome in children with septic shock receiving standard care.36 Other chemokines relevant to the pathophysiology of septic shock include monocyte chemoattractant protein 1 (MCP-1) and macrophage inflammatory protein-1 (MIP-1).34
Macrophage Migration Inhibitory Factor
Macrophage migration inhibitory factor (MIF) has recently emerged as an important cytokine in the pathophysiology of sepsis, and high levels of MIF in patients with septic shock and ARDS correlate with poor outcome.37,38 MIF possesses a number of biological activities generally directed toward a proinflammatory phenotype, including skewing of naive T cells toward a Th1 phenotype. An unusual feature of MIF is that its secretion is enhanced by glucocorticoids, whereas the expression and activity of many cytokines are suppressed by glucocorticoids. In turn, MIF has the ability to antagonize the antiinflammatory effects of glucocorticoids.
Interleukin-18
Interleukin-18 has also recently emerged as an important cytokine in the pathophysiology of sepsis.39 Depending on the local cytokine milieu, IL-18 has the ability to skew naive T cells toward either a Th1 or Th2 phenotype. In addition, it appears that IL-18 may serve as an early biomarker to distinguish between gram-positive and gram-negative sepsis.
Interleukin-10
Interleukin-10 is the best studied and most well known antiinflammatory cytokine.40,41 As an antiinflammatory cytokine, IL-10 serves to antagonize the proinflammatory effects of other cytokines and can thereby keep inflammation “in check.” IL-10 inhibits expression of cytokines such as TNF-α, IL-1β, and IL-8, and can inhibit expression of adhesion molecules. In addition, IL-10 can “deactivate” monocytes by downregulating the expression of MHC surface molecules. Thus IL-10 has a number of interesting properties that could potentially be leveraged therapeutically to limit excessive inflammation during sepsis. This theoretical consideration must be tempered by the ability of IL-10 to deactivate monocytes and thereby potentially impair the ability to adequately clear infection (i.e., the immune suppression paradigm depicted in Figure 103-1). Indeed, it has been reported that in children with multiple organ dysfunction syndrome, higher plasma IL-10 levels correlate with higher mortality, and higher monocyte mRNA levels of IL-10 correlate with increased length of stay in the ICU.42 Similar observations have been reported in adult patients with septic shock.43
High-Mobility Group Box 1
High-mobility group box 1 (HMGB-1) has long been known as a nonhistone DNA-binding protein. More recently, it has been recognized that HMGB-1 also exists in the extracellular compartment, appears to have proinflammatory properties that may play a role in the pathophysiology of sepsis, and may represent a potential therapeutic target for sepsis.44 The attraction of HMGB-1 as a therapeutic target in sepsis stems from the observation that it may be a “late mediator” of sepsis in as much as it appears in the extracellular compartment within a time frame that is considerably later than that seen with the canonical sepsis cytokines such as TNF-α and IL-1β. Thus the kinetics of HMGB-1 expression provide a potential therapeutic window that is clinically feasible to exploit. This temporal observation is evident in both experimental models of sepsis and in humans with established septic shock.45 The biologic properties of HMGB-1 appear to involve activation of toll-like receptors and the receptor for advanced glycation end products (RAGE). More recently, it has been suggested that HMGB-1 intrinsically possesses very little proinflammatory biological activity, but forms highly proinflammatory complexes with cytokines (e.g., IL-1β) and PAMPS (e.g., bacterial DNA and lipopolysaccharide).46
HMGB-1 is also a prime example of a class of molecules known as “alarmins” or danger/damage-associated molecular patterns (DAMPs).47 Broadly speaking, DAMPs represent a class of molecules that normally exist in the intracellular compartment at baseline, but are released from damaged cells into the extracellular compartment during conditions such as trauma or sepsis. DAMPs appear to signal through many of the same PRRs that recognize pathogens, and therefore have the ability to activate the immune/inflammatory system. Because DAMPs are released from damaged cells, they can serve to “alert” the inflammatory system of systemic “damage” or “danger” and can therefore induce appropriate and adaptive activation of defense mechanisms. Alternatively, excessive DAMP-mediated activation of PRRs can lead to unnecessary and maladaptive amplification of inflammation that is damaging to host tissues. Other examples of PAMPs include calgranulins, hepatoma-derived growth factor, heat shock proteins, and uric acid. In this regard, heat shock proteins have been reported to be substantially elevated in the serum of children with septic shock.48,49
Adhesion Molecules
An important breakthrough in the molecular understanding of sepsis-induced organ dysfunction came with the identification of the processes responsible for the infiltration of leukocytes into tissues.50 The “leukocyte-endothelial cell adhesion cascade” (Figure 103-2) is characterized by early cytokine-mediated activation of the selectin family of endothelial cell adhesion molecules that can mediate a process of neutrophil “rolling,” whereby sialyated moieties constitutively present on neutrophils interact with selectins on the endothelial cell membrane (e.g., E-selectin). In the second phase, activation of the “rolling” neutrophil causes increased expression and activation of the integrin family of adhesion molecules that interact with the similarly upregulated intercellular adhesion molecule (ICAM)-1 on the endothelial cell surface. This ligand interaction facilitates firm adhesion of the neutrophil to the endothelium. Subsequently, in response to a variety of chemotactic molecules, neutrophils transmigrate through the endothelial junctions to the site of inflammation. Release of a variety of radical species, both oxygen- and nitrogen-based, and proteases by the activated neutrophils can contribute to pathogen eradication, but paradoxically can also cause endothelial and tissue injury.
Nitric Oxide
Nitric oxide (NO) was discovered in the 1980s to be the molecule responsible for endothelial-derived relaxation of blood vessels.51,52 Since that time, NO has received tremendous attention as a potential mediator of septic shock.53 NO is produced by the enzyme nitric oxide synthase (NOS), which converts arginine and oxygen to NO and citrulline. Human NOS exists as three different isoforms (NOS 1, 2, and 3) and each isoform has relatively unique tissue localizations, requirements for NO production, and kinetics of NO production. Several features of NO-related biology support an important role in the pathophysiology of sepsis. First, NOS2 (also referred to as inducible NOS) is expressed in response to proinflammatory signals (e.g., lipopolysaccharide, TNF-α, and IL-1β) and produces large amounts of NO for prolonged periods of time. Second, NO can induce pathologic vasodilation and can function as a myocardial depressant. Third, NO can function as an oxidant either alone or by contributing to formation of other highly oxidizing, reactive molecules such as peroxynitrite. Fourth, NO has the potential to negatively affect mitochondrial function. Finally, elevated levels of NO metabolites have been well documented in children with septic shock and the levels correlate with the degree of cardiovascular dysfunction.54,55 Despite these intriguing biological properties, and a wealth of preclinical data testing the efficacy of NOS inhibition, clinical trials targeted at NOS inhibition have failed to demonstrate efficacy.56 Because NO has a myriad of biological properties important for maintenance of homeostasis (particularly when NO is produced by the NOS1 and NOS3 isoforms), this lack of efficacy may represent the timing and specificity of NOS isoform inhibition.
The Coagulation Cascade
It is now well established that the inflammatory cascade is directly linked to the coagulation cascade, and that the coagulation cascade can be pathologically activated in the context of sepsis.57,58 This pathological activation leads to disseminated intravascular coagulation, which subsequently leads to endothelial cell dysfunction and microvascular thrombosis. If the endothelial dysfunction and microvascular thrombosis progress to a critical threshold, end-organ failure ensues.
A complex network of multiple mediators takes part in this pathologic process, including proinflammatory cytokines, tissue factor, antithrombin III, protein C, protein S, tissue factor pathway inhibitor, and plaminogen activator inhibitor type 1 (PAI-1). Increased PAI-1 levels are a particularly strong feature of severe cases of meningococcemia and may be causally linked to a polymorphism in the promoter region of the PAI-1 gene.59,60 Depressed levels of the endogenous anticoagulants, antithrombin III, protein C, and protein S are consistently documented in the context of septic shock. These observations have led to multiple clinical trials in which recombinant forms of these endogenous anticoagulants have been administered to patients with septic shock. Thus far the only strategy that has been shown to reduce mortality is activated protein C (APC), which now has Food and Drug Administration (FDA) labeling specifically for adult patients with septic shock.61 The beneficial affects of APC in septic shock are thought to be secondary to both prevention of microvascular thrombosis and an antiinflammatory effect.62 Unfortunately, a Phase III trial of APC therapy in children with septic shock (the RESOLVE trial) failed to demonstrate efficacy.63 The RESOLVE trial was terminated after the second interim analysis due to little chance of reaching the primary efficacy endpoint. In addition, there was an increased risk of serious bleeding events in patients younger than 2 months of age. Nonetheless, the RESOLVE trial represents the largest and best-organized pediatric septic shock trial to date and provides an important context and reference point for all future interventional trials in the field of pediatric critical care medicine.
Related to the paradigm of altered coagulation playing an important role in the pathophysiology of sepsis is the concept of thrombocytopenia-associated multiple organ failure (TAMOF).64 New-onset thrombocytopenia in critically ill patients, including patients with sepsis, correlates with the evolution of persistent organ failure and poor outcome. The mechanistic link between thrombocytopenia and organ failure is thought to involve a form of microangiopathy analagous to thrombotic thrombocytopenic purpura, involving substantial decreases of ADAMTS-13 (A Disintegrin and Metalloprotease with ThromboSpondin motifs). ADAMTS-13 regulates microvascular thrombosis by cleaving the large thrombogenic von Willebrand factor multimers into smaller, less thrombogenic forms. Prelminary experience indicates that plasma exchange restores ADAMTS-13 levels and restores organ function in children with TAMOF.65 The future conduct of large, multicenter, randomized trials will be imperative to objectively establish the potentially exciting role of plasma exchange in this patient population.
The Peroxisome Proliferator-Activated Receptor-γ Pathway
The peroxisome proliferator-activated receptor-γ (PPARγ) is a member of the PPAR nuclear receptor superfamily and a ligand-activated transcription factor having well-known effects on lipid metabolism and cell proliferation.66 The thiazolidinedione class of insulin-sensitizing drugs are well known PPARγ ligands (activators) and are currently widely used in the management of type II diabetes. Recently, it has become evident that pharmacologic activation of PPARγ has important antiinflammatory effects that are of significant benefit in experimental models of critical illness, including sepsis.67–71 The recent demonstration that PPARγ expression and activation is indeed altered in children with septic shock, coupled with the availability of FDA-approved PPARγ ligands, opens up the realistic possibility of conducting clinical sepsis intervention trials focused on PPARγ activation in the near future.72
The Paradigm of Sepsis as an Adaptive Immune Problem
Our conceptual framework of the pathophysiology of septic shock has evolved over the last decade to include the concept of immune paralysis. Whereas septic shock has been traditionally viewed as being a reflection of uncontrolled hyperinflammation (i.e., an innate immunity problem), it is now thought that septic shock also has a strong, perhaps predominant, “antiinflammatory” component that can be manifest as immune suppression and the relative inability to effectively clear an infectious challenge (an adaptive immunity problem).16,17,73,74 For example, monocyte deactivation related to decreased major histocompatibility complex (MHC) gene mRNA expression and decreased surface expression of MHC molecules have been previously demonstrated in patients with septic shock, including children.42,43,75,76 With regard to lymphocyte dysfunction, Heidecke and colleagues demonstrated that adult patients with intraabdominal infections and septic shock have defective T cell proliferation and defective T cell–dependent cytokine secretion, all of which is consistent with anergy/immune suppression.77 Felmet and colleagues identified prolonged lymphopenia and apoptosis-associated depletion of lymphoid organs as independent risk factors for the development of nosocomial infections and multiple organ failure in critically ill children.78 Animal studies have well documented the requirement of an intact T-cell system to adequately combat a septic challenge.74,79 More recently, animal-based experiments have demonstrated that experimental septic shock is characterized by widespread apoptosis of T cells, and that preventing T-cell apoptosis positively impacts the outcome of experimental sepsis.80–87 Importantly, the concept of T-cell apoptosis in human sepsis has been indirectly corroborated by autopsy studies, including children,78,88 and lymphocyte-based immunophenotyping was recently demonstrated to effectively stratify septic shock outcome in adults.89 Finally, it has been recently demonstrated in experimental models that alterations of the adaptive immune system in sepsis can persist well beyond the acute period (up to at least 6 weeks) via epigenetic mechanisms affecting dendritic cells.90,91 Despite these data, formal studies of T-cell function and adaptive immunity in pediatric septic shock have never been conducted in a systematic and comprehensive manner. Such studies hold the promise of radically changing our conceptual approach to the long sought, but not yet realized, goal of rational immune modulation in septic shock.
Genomic Medicine and Sepsis
Genetic Influence and Septic Shock
Susceptibility to sepsis and the clinical course of patients with sepsis are both highly heterogeneous, thus raising the strong possibility that the host response to infection is, at least in part, influenced by heritable factors (i.e., genetics).92 A landmark study by Sorensen et al., published more than 20 years ago, provides strong evidence linking genetics and susceptibility to infection.93 This study involved a longitudinal cohort of more than 900 adopted children born between 1924 and 1926. The adopted children and both their biologic and adoptive parents were followed through 1982. If a biologic parent died of infection before the age of 50 years, the relative risk of death from infectious causes in the child was 5.8 (95% confidence interval, 2.5 to 13.7), which was higher than for all other causes studied, including cancer and cardiovascular/cerebrovascular disease. In contrast, the death of an adoptive parent from infectious causes did not confer a greater relative risk of death in the adopted child.
More recently, investigations attempting to link genetics with sepsis have largely focused on candidate-gene association studies and gene polymorphisms. A gene polymorphism is defined as the regular occurrence (>1%) in a population of two or more alleles at a particular chromosome location. The most frequent type of polymorphism is called a single nucleotide polymorphism (SNP): a substitution, deletion, or insertion of a single nucleotide that occurs in approximately 1 per every 1000 base pairs of human DNA. SNPs can result in an absolute deficiency in protein, an altered protein, a change in the level of normal protein expression, or no discernible change in protein function or expression. There is a growing body of literature linking SNPs within several genes that regulate inflammation, coagulation, and the immune response with critical illness, and several excellent reviews exist on the topic.94–97
The signaling mechanisms involved in pathogen recognition, the immune response, and inflammation were described in previous sections. Herein, we will provide an overview of relevant SNPs that have been described in many of the genes involved in these signaling mechanisms. TLR-4 (the primary receptor for recognition of lipopolysaccharide) mutations have been described in humans, all of which increase susceptibility to infections secondary to gram-negative organisms.98 While several SNPs in the TLR-4 receptor gene have been described, few have been found to be associated with an increased risk of septic shock or septic shock-related mortality in children. For example, an adenine for guanine substitution 896 base pairs downstream of the transcription start site for TLR-4 (+896) results in replacement of aspartic acid with glycine at amino acid 299 (Asp299Gly). The Asp299Gly polymorphism has been associated with reduced expression and function of the TLR-4 receptor in vitro.98,99 Furthermore, adults who carry the Asp299Gly polymorphism appear to be at increased risk for septic shock and poor outcome in several cohort studies.100–102 While children who carry the Asp299Gly polymorphism appear to be at increased risk of urinary tract infection, this SNP does not appear to influence either the susceptibility or severity of meningococcal septic shock in children.103,104 These results were further corroborated in a cohort study involving over 500 Gambian children.105
SNPs related to other members of the LPS-receptor complex (e.g., CD14, MD-2, and MyD88) have been studied in adult populations, but no such studies have yet to be performed in children.101,106–109 SNPs in other classes of toll-like receptors have also been studied. For example, gene polymorphisms of TLR-2, the primary pattern recognition receptor for gram-positive bacteria, have been associated with increased risk of infection in children and adults.110–112
Several SNPs affecting cytokine expression have been described, but the corresponding gene association studies in critically ill adults with septic shock have been conflicting.96,97,113 For example, two allelic variants of the TNF-α gene have been described: the wild-type allele TNF1 (guanine at –308A), and TNF2 (adenosine at –308A). The TNF2 allele has been associated with higher expression of TNF-α and increased susceptibility to septic shock and mortality in at least one study involving critically ill adults.114 Nadel and colleagues found an increased risk of death in critically ill children with meningococcal septic shock who carried the TNF2 allelic variant.115 Several additional SNPs in TNF-β, IL-1, IL-6, IL-8, and IL-10 have also been shown to influence susceptibility to and severity of septic shock in children.116–122
Because dysregulation of the coagulation cascade plays an important role in the pathophysiology of septic shock, several studies have examined polymorphisms of key genes involved in coagulation. For example, the 4G allele of a deletion/insertion (4G/5G) SNP in the promoter region of the plasminogen-activator inhibitor type-1 (PAI-1) gene has been associated with higher plasma concentrations of PAI-1. The 4G allele increases susceptibility to and severity of septic shock, as well as increasing the risk of mortality in children with meningococcal septic shock.59,123–125 In addition, an SNP in the protein C promoter region has been associated with susceptibility to meningococcemia and illness severity in children.126
SNPs in genes involved in phagocytosis and the complement cascade have also been studied in the context of septic shock. For example, SNPs that affect function have been described in virtually all family members of the Fcγ receptor (important for phagocytosis), and several of these SNPs have been associated with susceptibility to meningococcal sepsis, severity of meningococcemia, and poor outcome from meningococcal septic shock.127–133 In addition, an association between the FcγRIIa polymorphism and infection with other encapsulated bacteria has also been reported.134,135 Several SNPs in the mannose-binding lectin (MBL) gene have been associated with increased susceptibility to infection, as well as increased illness severity.136–141 Finally, an SNP of the bactericidal/permeability-increasing protein (BPI) gene has also been associated with increased mortality from septic shock in children.142 This polymorphism is particularly interesting given that a well-conducted Phase III trial of recombinant BPI in children with septic shock failed to demonstrate efficacy.143
It is likely that many more studies are forthcoming in the future that will attempt to link SNPs with the susceptibility and/or outcome of pediatric septic shock, and all need to be carefully considered and evaluated. With respect to validity and wide clinical acceptance, the ideal candidate-gene association study requires several important qualities including biological plausibility, large sample sizes, a priori hypothesis statements and power calculations, accounting for confounding factors, and independent validation.144
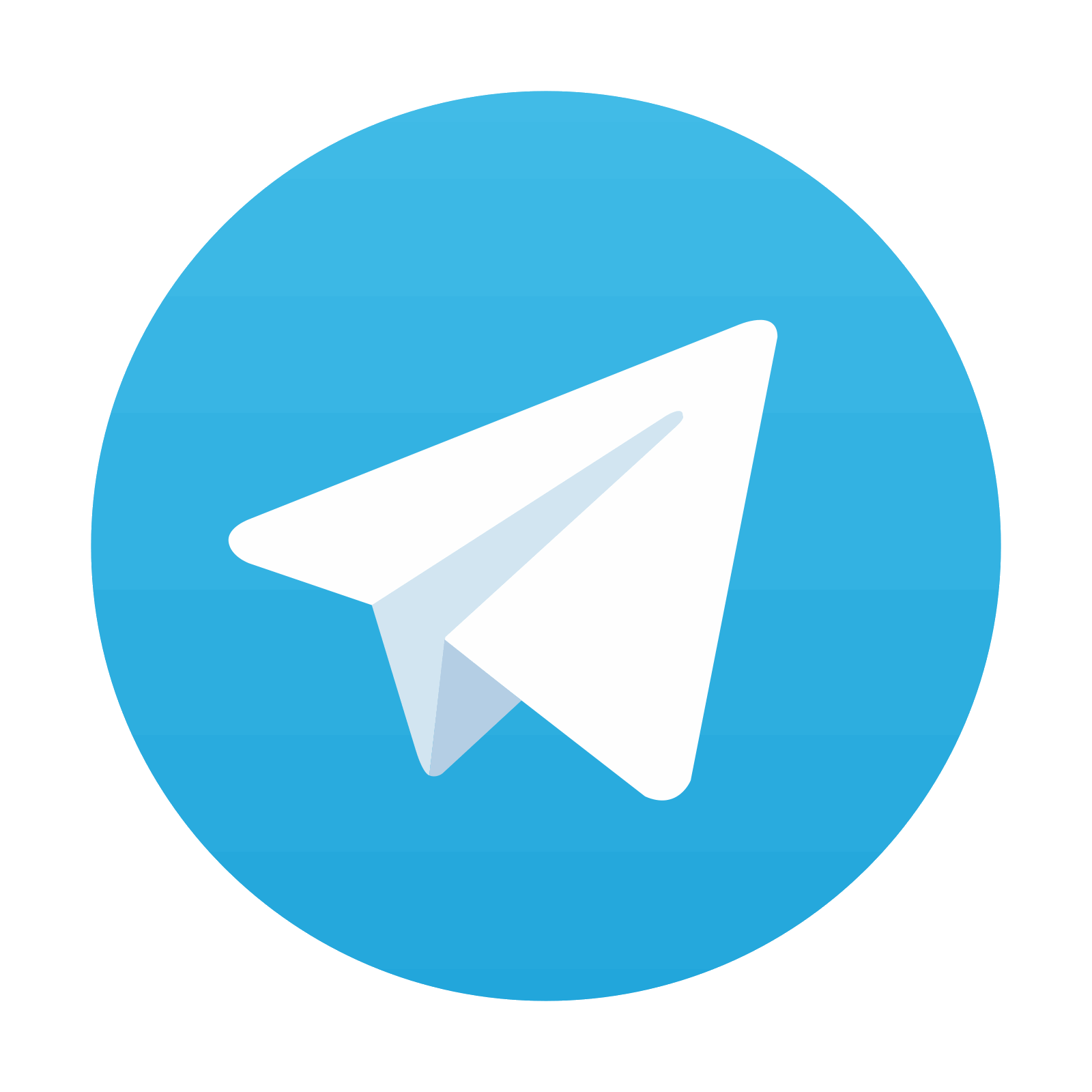
Stay updated, free articles. Join our Telegram channel
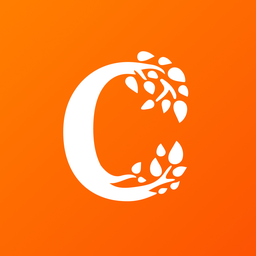
Full access? Get Clinical Tree
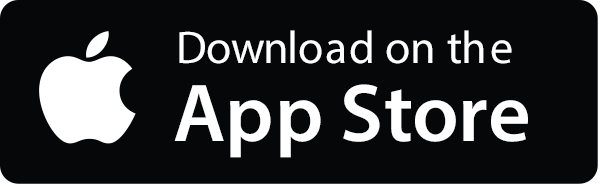
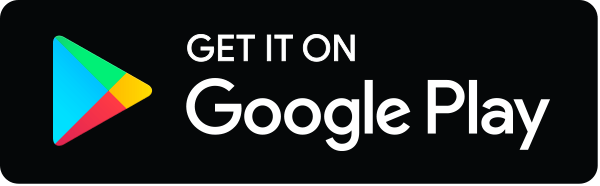