191 Resuscitation of Hypovolemic Shock
Epidemiology of Severe Hemorrhagic Shock
Mechanisms of injury and severity of blood loss as well as prehospital interventions vary widely among trauma centers. Preferred fluid resuscitation strategies and optimal blood pressures are still being studied.1,2 The number of preventable deaths due to hemorrhage are still significant. Definitive control of hemorrhage and resuscitative strategies are the cornerstone of treatment.3
Current State of Knowledge About Inadequate or Incomplete Resuscitation in Hemorrhagic and Hypovolemic Shock
Cardiovascular and Hemodynamic Response
Shock is defined as inadequate delivery of O2 to metabolically active tissues. Failure of O2 delivery can lead to eventual organ dysfunction and ultimate complete circulatory collapse. Guyton described three major stages describing the mechanisms.4 First is compensated shock, in which the individual will achieve full recovery with minimal interventions. Regional tissues and organs have different mechanisms to prevent damage. The next stage is decompensated shock. Aggressive resuscitation is required in this stage, or a substantial fraction of individuals will die. There is a poor correlation between changes in cardiac output and systemic blood pressure. Irreversible shock is the last stage. Shock has progressed to the point that all known therapies are inadequate.
< div class='tao-gold-member'>
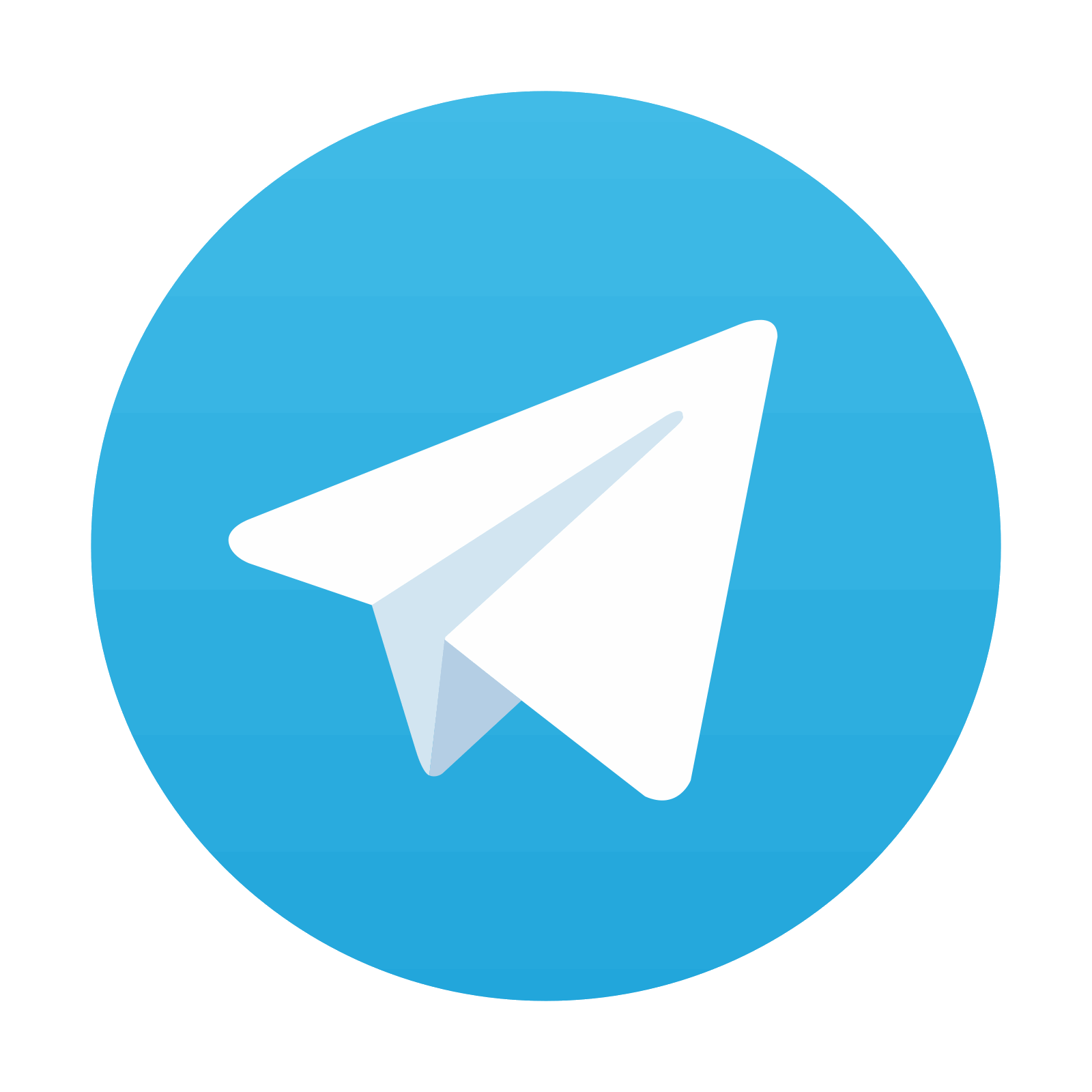
Stay updated, free articles. Join our Telegram channel
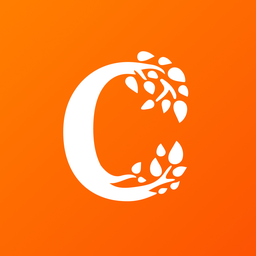
Full access? Get Clinical Tree
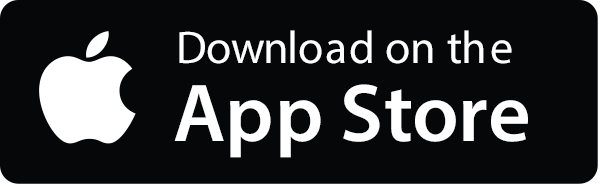
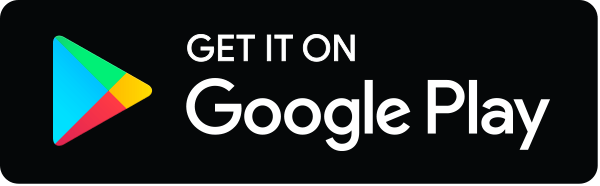