Chapter 4 Research in Pediatric Critical Care
Research Areas
There are clearly pathophysiologic processes that appear to be unique to critical care medicine. Probably the most clear-cut of these processes is acute respiratory distress syndrome (ARDS),1,2 although this is only one manifestation of a systemic process. The syndrome appears to develop in critically ill patients whose initial injury arises from a wide variety of organ-specific insults. Although the lungs appear to be the primary target organ, all organs are affected by the same complex underlying pathophysiologic process. This process causes widespread endothelial injury involving many organ systems.3 This results in tissue edema, decreased organ perfusion, ischemia, and multiple organ failure. This pansystemic disease is familiar to the intensivist and is one of the most extensive areas of unique research interest in critical care today. Understanding this systemic inflammatory response is central to understanding critical care.
Another major disease process that clearly interests critical care physicians is shock. Shock, whether it be hypovolemic, septic, or of some other etiology, by its very nature is a multisystem, non–organ-specific, acute, life-threatening process.4,5 Another natural area for critical care research would be specifically aimed at preventing and/or ameliorating the multisystem insults that occur because of the body-wide activation of potentially lethal mediators triggered by episodes of shock. The spectrum of research opportunities in this area ranges from molecular biologic to large-scale clinical trials and has grown exponentially in the past few years.6
Organ system interaction also provides an area of primary interest in critical care. In particular, cardiorespiratory interaction has immediate, everyday application in ventilatory management, cardiopulmonary resuscitation, and cardiac support. The physiology of how changes in the pleural pressure affect cardiac function during either spontaneous or positive pressure ventilation is an area of constant relevance to the intensivist.7 In a broader sense, cardiorespiratory interaction extends beyond this arena. How changes in cardiac function alter ventilation, airway resistance, and lung compliance is an integral part of critical care. The pulmonary endothelial synthesis, release, and degradation of multiple mediators, ranging from myocardial depressant factors to systemic vasoactive substances, that alter cardiovascular function can be considered cardiorespiratory interaction and are of unique interest to the intensivist.7 This area also encompasses a broad spectrum of possible approaches from molecular biology to cell physiology to integrated physiology. An area of research that is particularly important to critical care medicine is cell biology. All organ system failure can be described in terms of cellular failure. For example, the general effects of superoxide radicals produced by leukocytes on other cell functions are legion and clearly of interest to intensivists.4,8 Extending the argument of cellular specialization is possible for other cell types. An argument could be made to consider the endothelium the specialty organ of intensivists.9 All organs that fail in critically ill children contain large areas of biochemically active endothelium. This endothelium is important because it elaborates hormones and autacoids that have systemic and local effects. These effects include alteration of coagulation and blood viscosity, superoxide generation and tissue damage, smooth muscle regulation, metabolism of circulating vasoactive substances, and interaction with the immunologic system.10,11 All generalized stressors (e.g., sepsis, hypoxia, hypovolemia) alter endothelial cell function. One way of looking at multiple organ system failure is to view it as an “endotheliopathy.” This endotheliopathy gives rise to widespread organ-specific damage, such as renal failure, ARDS, myocardial depression, and alterations in the blood-brain barrier, with subsequent cerebral edema. Endothelial cell function changes over time as the child develops and may be specifically affected by disease processes particularly prevalent in children, such as the infectious vasculitides.12 This sort of theoretical construct could serve as an organizational basis for research efforts in pediatric critical care and offer new insights into our understanding of critical illness in children.
Because of the astonishing success of the human genome project, the development of gene chips, and the increasingly realized potential of proteomics, understanding the genetic nature of critical illness no longer seems beyond our reach.13 Understanding the genotype of individuals who physiotypically display fatal responses to meningococcal disease while their classmates remain asymptomatic, albeit colonized by the same serotype organism, holds the promise of prospectively tailoring therapy to each patient individually. The many genomic and proteomic projects developing within pediatric critical care will help our understanding of the genetic bases of critical illness and holds out hope for understanding systemic inflammatory response syndrome (SIRS) and sepsis.13–15
Finally, and perhaps most importantly, the burgeoning area of medical informatics holds the promise of enabling us to understand complex, critical, but rare disease processes previously impossible to study.16–19 Understanding knowledge discovery in databases and building national and international collaborative partnerships to understand the scope of pediatric critical care have come within our reach.20 Informatics research with national funding may provide real-time guidance for management of the rarest critical illnesses. In addition participation in national databases to support quality improvement and multisite research studies provide a new and fertile area for critical care research.21,22
These examples indicate part of the wide spectrum of research opportunities in pediatric critical care medicine. There are many more, including the now well-established multicenter National Collaborative Pediatric Critical Care Research Network, now in its second 5-year epoch of funding by the National Institute of Child Health and Human Development (NICHD), one of the National Institutes of Health. Networked research is necessary in critical care and the Pediatric Lung Injury and Sepsis Investigators (PALISI; http://pedsccm.org/PALISI_network.php) is an important example of a national research network focused on pediatric critical care. Constant sensitivity to identifying the questions (incorrectly answered, unanswered, and unasked) is the character trait required in the academic intensivist if our specialty is to continue to grow. Identifying areas unique to critical care medicine provides the knowledge base for the specialty necessary for its growth and for our patients’ well-being. Given the many unique areas of interest in critical care, how do we uncover them and encourage research in the subspecialty?
Wellsprings of Research
Collective Needs
Why must critical care collectively, as a specialty, support research in pediatric critical care? The arguments mentioned previously suggest that without the academic, intellectual, and scientific pursuit of areas that are specifically unique and relevant to critical care, we have no specialty. In this broad sense, research establishes a collegial respect for physicians who practice critical care and engenders academic support for the specialty. If all we do is provide clinical care for desperately ill or dying children, and clinical care that other physicians do not understand by virtue of their not being dedicated to it, the challenge of “what do intensivists do?” should only in part be answered by “spend long hours with children who are critically ill and their families.” This question addresses a deeper issue: Are intensivists contributing to the further understanding of critical illness? Are intensivists contributing to the intellectual advancement of medicine and the rich intellectual and academic milieu in the universities in which they find themselves? Are intensivists obtaining extramural funding for university-wide interactive and collaborative research efforts? Are intensivists training future generations of physician-scientists? Unless we can affirmatively answer these questions from a uniquely critical care point of view, it should not be surprising that physicians in other subspecialties do not understand or reliably value our labors. This justification—to ensure the viability and respectability of a specialty whose primary concern is treating the critically ill—is a major reason we must encourage and support research. Failure to do so is a failure to critically ill children.
Individual Motivation
Personal motivation is the necessary starting point for research invovlement. No amount of external pressure can produce a successful researcher. Where does this personal motivation spring from? The noble goal of adding to the knowledge base of the field is excellent; however, it is unlikely that many clinicians wake up in the morning and say, “Aha! I will add to the knowledge base of critical care today.” In the practice setting clinicians are continually challenged by questions that arise from providing critical care for children, by questions about patient management, and by uncertainties and confusion in providing patient care. It must be very rare indeed for a young clinician not to wonder about, be curious about, or be interested in answering these questions. It is the role of all preceptors in critical care to make certain that the trainee is aware of these questions and that they are asked. To teach critical care as if it were dogma is destructive to these goals. To constantly point out and demonstrate where there are failures and conflicts in our understanding and where matters of style, rather than matters of substance, determine our clinical practice is to uncover fertile areas for research. Unless the young intensivist senses these exciting challenges, the personal enthusiasm toward research will not be discovered.
The previously mentioned personally motivating factors, which include personal aggrandizement such as fame, fortune, job security, avoidance of burnout, fun, ability, education, and training, still are not, however, sufficient. All of these possible motivations do not provide the major essential driving force. Individual curiosity, a tireless need to question, and the restless search for answers must be the source of the entire endeavor. Curiosity? Is this the crucial concept? Sir Peter Medawar calls it a “nursery word”—a motive too inadequate.23 Everyone possesses curiosity, and yet not everyone makes a commitment to seek solutions, occasionally at great personal cost. So it must be more than mere curiosity. Medawar calls this driving compulsion the “exploratory impulsion”; Kant called it “restless endeavor.”23 It is not merely curiosity but a surrender to the urge, often sacrificially, to seek the answer that motivates the investigator. This urge must be strong because it will require a great deal of time and energy before the question that originally piqued the clinician’s curiosity can be addressed. This innate, compelling motivation of the individual is the main driving force of medical investigation.
Where the collective needs of the specialty and the individual’s needs come together is that both have a genuine, deep-rooted desire to understand better how to help critically ill patients. This symbiosis of specialty needs and individual motivation forms the essential chemistry of discovery. In a fascinating address to the American Society for Clinical Investigation, J.L. Goldstein24 presented the formula:
Let us not train too many fools. Constantly striving to recruit the seeker, doubter, questioner—and when they are recruited, to support them—is the responsibility of all physicians involved in critical care. Without them, critical care research will be nonexistent. Significant advances in our specialty can grow only from accepting this responsibility. Without this commitment to encourage and train, critical care medicine will lose promising young clinician-scientists to other specialty areas, because it will be only there that they will be able to seek answers to their questions. This crucial issue and its centrality to the growth of our specialty cannot be overemphasized. The training also must be thorough. This takes time, but without the commitment to train young investigators to think like basic scientists, they will be unable to apply the tools of basic science and will end up paralyzed and lost to the specialty of pediatric critical care.24
Doing Research
So, what is research? Research is scientific investigation. If the motivation to do research can be matched by the commitment of the specialty to support research, is that sufficient? How is the bedside problem answered? If a keen investigator with a good question has a willing pediatric intensive care unit director with money, or at least one who is willing to help find resources, what next? How is research done? What is the scientific process?
Over the past 200 years, the scientific method has been developed by learning how to test our guesses about the universe.25,26 The key factors involved are the following:
What is an experiment? The original meaning of the term experiment was “a test made to demonstrate a known truth.” It served as a means of proof for an already “known” truth. This sort of experiment was not designed to generate new knowledge. This Aristotelian concept of experimentation, involving classical deductive logic, has limited application in modern medicine, other than perhaps that of pedantry and teaching high school chemistry. The essential concept of experimentation that has a more contemporary meaning entails an uncertain or unknown outcome. To the present-day researcher, the purpose of performing an experiment is to discriminate between possibilities. How experiments are designed to discriminate between possibilities depends on the underlying assumptions. Understanding the logic that underlies how an experiment is performed is useful in avoiding multiple traps, not only in reviewing the data but also in applying experimental results to real patients. Bacon27 noted: “If a man begins with certainties, he shall end in doubts, but if he will be content to begin with doubts, he shall end in certainties.” Many experiments are still designed—contrived may be a better word—to demonstrate the validity of preconceived ideas (sometimes called an ‘hypothesis’). This reasoning from preconceived ideas or premises to the specific situation is known as the process of deductive logic. Deductive logic is reasoning from the general to the specific. A clinical example of such deductive logic is the following syllogism:
The difficulty comes when the dogmatic assumptions that underlie our clinical practice, and deductively lead to our therapies, are incorrect. Aristotelian experimentation provides no way to approach outcomes in medicine that are exceptional, yet these are the very occurrences that may be enlightening. Charles Darwin has exhorted us never to allow these exceptions to go unnoticed.28 Deductive logic is unable to assist in the discovery of new knowledge and therefore general principles. This was clearly noted by Bacon,29 who stated in 1620:
The great revolution in scientific and philosophic writing in the 1600s was typified by Bacon’s absolute refutation of the concept that any new truths could be discovered merely by a deductive act of the mind4:
A clinical example of this sort of contribution to modern medicine is the well-known example of vaccination. Jenner’s recurrent observation of the specific immunity to smallpox of patients who had been infected by cowpox led to experimentation with observation and a series of inductive steps that ultimately led not only to the eradication of smallpox in the world but also to generalization of the concept of vaccination to other infectious processes and vast discoveries in the area of immunology. It is impossible to conceive how Aristotelian deductive logic could have led to these discoveries. Bacon’s contribution was to realize that observations of the specifics in nature would lead, through application of the intellect, through inductive logic, to the discovery of new truths. Bacon did realize that we were unable to rely on “the casual felicity of particular events”23 to provide us with all the specific information required to discover scientific truths, even if we spent an entire lifetime observing nature. He thus realized the necessity to devise experiences and contrive occurrences to collect factual information by which we would understand the natural world. This is Baconian experimentation. However, this still was not experimentation as practiced in medicine today.
Current medical experimentation is more accurately described as Galilean experimentation.23,28 The Galilean experiment discriminates between possibilities and, in so doing, confirms a preconceived notion by supplying facts that support the inductive process and lead to a sound conclusion. The essence of an experiment as proposed by Galileo was a true test, a trial, or an ordeal of an hypothesis. This constructive experimentation more accurately reflects what we think of today when we want to further our understanding of critical illness. As Stephen Hawking26 put it in A Brief History of Time:
This syllogism was disproved by a single observation. Therefore the conclusion was incorrect and therefore if the minor premise was true, the major premise had to be false. A new intellectual universe became possible. Every preconceived notion was testable by experiment. All that is required is a testable hypothesis. The routine function of medical experimentation, the purpose of all medical science and the major modus vivendi of all the national institutes, is the testing of hypotheses. Although gathering facts and cataloging their relationships as in Aristotelian and Baconian experimentation remains of some value, testing hypotheses is our strongest research tool. Galilean experimentation provided the capability of constantly revising our hypotheses and avoiding unnecessary persistence in theoretical structures based on hypothetical errors that lead to no more than a house of cards.The generation of scientific hypotheses that can be critically tested is the basis of scientific discovery. Discovery has its beginnings in imaginative preconception, which is the creative act of mind that gives rise to a hypothesis.28 Asking the question or conceiving the question is only the beginning of the scientific process. Casting the question in the form of testable, verifiable, scientific hypotheses is where the creative work of research really begins. The brilliant guess, the eureka moment—these scientific insights are the sources of these hypotheses. The hypothesis is a mark to be attained, a suggestion of the probable, a provisional proposal of an underlying truth, or some specific facet of it. The hypothesis has but one purpose, to be tested. But this approach means that an hypothesis, no matter how interesting, can never be proven. Absolute proof of a hypothesis is not, by the very nature of inductive logic and Galilean experimentation, ever possible because all possibilities cannot possibly ever be tested. Again, according to Stephen Hawking26:
Whereas a theory is an organized system of knowledge used to analyze or explain nature or behavior, a hypothesis has no such value. Theories may be built up from facts learned by testing hypotheses and may even contain partially substantiated hypotheses that are useful in predicting events, but hypotheses are useful only insofar as their testing acts as a focus for the discovery of truths. Without a doubt, hypotheses are the most important instruments in research. Developing a hypothesis is the initial phase of research and scientific investigation. It generates the plan for the research. Nevertheless, it is “a means, not an end,” as Thomas Huxley cautioned.28 The ultimate goal of research is not to hunt blindly for unrelated facts but to test related hypotheses.
If we accept the fact that the purpose of experimentation is to test hypotheses, then it is clear that a necessity for research is to formulate appropriate hypotheses. The hypothesis must be focused, with a limited number of possible outcomes and limited number of implications that lead logically to further investigational steps. This minimizes futile activity. A hypothesis that accommodates all possible phenomena or outcomes is totally uninformative. The more restrictive it is, the more focused it is, the more instructive it is. One final warning about hypotheses: although they are the driving force of research, they must be kept in their place. Accepting unproved hypotheses can clearly lead you down a rabbit hole, often a time-consuming, expensive, and disastrous one. Failure to give up unsubstantiated or disproven hypotheses can lead (and has led) to a futile cycle of experimentation. Although scientists require hypotheses, find them attractive, and may not be able to live without them, they must not fall in love with them.23,28 The basic fact of science, that hypotheses are never proven and that they are only as good as the results they generate, must never be forgotten. Likewise, the physician-scientist as observer of nature must be encouraged to use quantitative and qualitative descriptive tools to develop the platform for meaningful hypothesis generation and testing.
Medical Research
Clinical research can be either retrospective or prospective. Retrospectively, epidemiologic studies, demographic studies, and studies of disease processes and outcomes of management regimens can provide useful information in directing future therapy. Certainly the great wealth of data now available in patient records can continue to provide worthwhile insights to aid our patients. Unfortunately, retrospective trials cannot convincingly answer therapeutic questions; rather, their utility is in hypothesis generation. Their solution requires true Galilean experimentation. Baconian studies such as these prospective trials require as much planning as possible before the patient actually is observed for the results of a therapeutic intervention, but this has started to change. Learning from reliable observations is the basis of physical science, and applying these research principles to data obtained from human subjects is useful in reliably suggesting a general theory if large enough numbers of observations are collected and analyzed. In medical informatics, this is the basis for knowledge discovery in databases. Thus with a sufficient number of observations (controlled, defined, verified data) we may learn how to manage our patients by applying analytical techniques to retrospective events. The information revolution may be driving knowledge discovery once again toward some reliance on deductive logic.18
The other overwhelming principle that guides clinical research is to preserve the rights, autonomy, and safety of the individual subject.30–32 This is of particular importance in clinical research involving children. The issues of risk, informed consent, and the potential to benefit the patient are particularly finely focused in pediatrics. The spectrum of opinion runs from believing that research in children is not allowable to believing that child subjects should be treated exactly the same as adult experimental subjects. Any researcher who proposes doing clinical research in critically ill children must be familiar with all aspects of these arguments and realize the sensitivity of the issues involved in this area.33,35
Dependent Variable
Most statistical analyses limit themselves to assessment of one dependent variable at a time. Selection of the dependent variable is determined by its distribution within the population, how reliably it can be measured, how sensitive and specific it will be to the independent variable, and how practical it is to measure. Clearly, maximum sensitivity and reliability are preferable. The more sensitive and reliable the selected dependent variables, the more likely the time and effort invested, number of subjects required, and cost of investigating the hypothesis will be minimized. Variables may be either quantitative or categorical—nonnumeric and discontinuous. With regard to distribution, it is generally assumed that dependent quantitative variables in the study population will undergo a normal (gaussian, bell-shaped curve) distribution. When abnormal distribution occurs, it must be specifically addressed statistically. It also is possible, in some instances, to transform an abnormal distribution to a normal distribution for the purposes of analysis. Disease states are often not normally distributed and parameters may be skewed (asymmetric) or demonstrate kurtosis (distribution of values near the mean) both of which alter how the population variance relates to the mean and therefore the validity of statistical inferences. Unfortunately, a single dependent variable rarely approximates the clinical situation, where a single independent variable intervention may have a series of effects on a host of dependent variables. Therefore it is frequently necessary to evaluate two or more dependent variables at any given time. This process requires advanced study design and analysis that takes requirement into account; for example, multivariant analysis may be required.
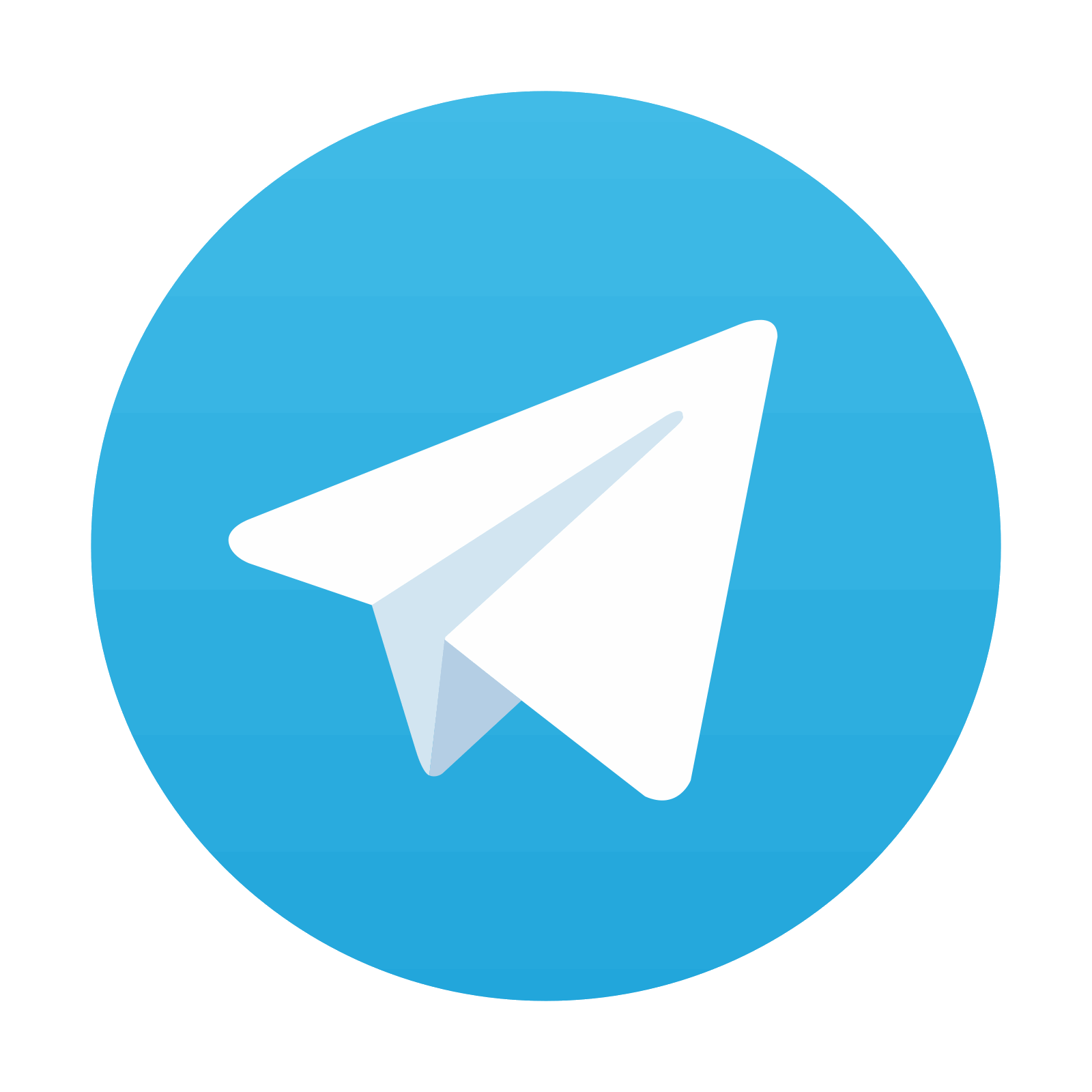
Stay updated, free articles. Join our Telegram channel
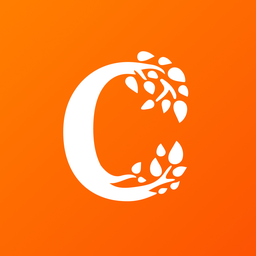
Full access? Get Clinical Tree
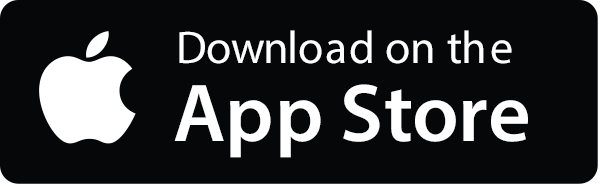
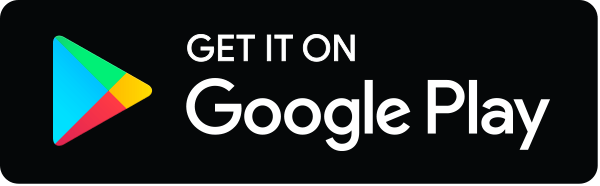