Chapter 66 Renal Structure and Function
Renal Development
The human kidneys begin development in the third week of gestation, at which time they are primitive organs called pronephroi. These early kidneys are functional but regress as development unfolds. As gestation continues in the fourth week, the secondary kidney elements, the mesonephroi, form from parallel strips of mesoderm along the paravertebral axis. The mesonephroi begin functioning between the sixth and tenth week of gestation before involution in a cranial-caudal direction beginning at 10 weeks’ gestation. The definitive kidney, or metanephros, begins development at the fifth week of gestation and begins functioning between the tenth and fourteenth week. This kidney develops in the pelvis as the branching ureteric bud and undifferentiated metanephric mesenchyme interact in a complex series of reciprocal inductions.1–3 These interactions lead to the formation of glomeruli, whereas vessels and tubules form from mesenchymal precursors, and distal tubules and collecting ducts derive from ureteric bud epithelium. This process occurs in a centrifugal fashion so that deeper corticomedullary nephrons form earliest in organogenesis, whereas the more peripheral cortical nephrons form later. As the metanephros develops, the maturing kidney ascends into the retroperitoneal space to its final location with the upper poles at the T12 vertebra. During the ascent, the systemic blood supply is derived from more cranial aspects of the aorta and from the lumbar renal arteries at the final position of the kidney. The ureters elongate and canalize during the ascent to maintain drainage to the bladder. By the time human nephrogenesis is complete at 34 to 36 weeks of gestation, repeated cycles of mesenchymal induction, ureteric branching, and morphogenesis result in approximately 1 million nephrons per kidney.
Renal Anatomy
Normal human kidneys reside in the retroperitoneal space at the level of the T12 vertebra. The liver is superior to the right kidney and thus displaces it lower than the kidney on the left side. The spleen and stomach overlie the superior aspect of the left kidney. Kidneys, however, can be found in a variety of other locations and have altered morphologies as a result of alterations of the normal developmental program (reviewed by Schedl4). For example, failure of the kidney to ascend normally results in a pelvic kidney that has abnormal vascular supplies from the aorta and iliac vessels. Mesenchymal regions of the two kidneys coming in contact during early development likely cause fused kidneys, most commonly the horseshoe kidney. Partial or complete renal duplications comprise a variety of abnormalities that may arise from aberrant branching of the ureteric bud into the developing mesenchyme. Unilateral agenesis likely results from failure of ureteric bud development or abnormal mesenchymal induction, leading to regression of the metanephric mesenchyme and failed renal development.
Renal Vasculature
Vascular Development
Markers of early vascular development are expressed in undifferentiated metanephric mesenchyme, which suggests that the blood supply to the nephron develops at least partially from precursors inherent to the maturing kidney.5 Migration of committed endothelial cells into the developing glomerulus occurs in response to secreted factors such as vascular endothelial growth factor, which is secreted under the transcriptional regulation of the oxygen-sensitive hypoxic inducible factor.6 Control of the corresponding branching of extraglomerular vessels is an area of active study and may involve branching from existing vessels, de novo vessel formation, or both processes. These actions appear to be regulated in part by the renin-angiotensin system.7
Vascular Anatomy
Venous drainage of the vasa rectae is divided into two types: the vessels of the deep medulla ascend to join the arcuate veins at the corticomedullary junction, and those of the superficial medulla ascend into the cortex to join the cortical capillary network and ultimately the interlobular and arcuate veins (Figure 66-1). The arcuate veins join with the interlobar veins via the interlobular veins and finally drain into the main renal vein to join the main circulation.
Vascular Function
The kidneys are extraordinarily vascular organs; they receive 15% to 18% of cardiac output in the neonate and up to 20% of cardiac output in the adult.8 Blood flow to the kidney is tightly regulated to ensure continued renal function over a range of blood pressures. Sympathetic α1-receptors, myogenic contraction, and vasoactive mediators control vascular resistance and provide autoregulation of renal blood flow. Maintenance of glomerular filtration rate at the level of the glomerulus occurs by vasoconstriction of efferent arterioles in response to pressors such as angiotensin II, whereas afferent arterioles relax in response to vasodilators such as prostacyclin. Circulating angiotensin II is elevated in the neonate,9 as are corresponding vasodilators.10,11 Therefore infants have a decreased capacity to regulate renal blood flow, which explains their increased susceptibility to renal ischemia in hypotensive states.
The Nephron Unit
The nephron unit consists of a glomerular tuft, proximal tubule, loop of Henle, distal tubule, and collecting duct (Figure 66-2). The proximal tubule is an extension of the urinary space of the glomerulus and courses into the loop of Henle. Two types of nephrons are characterized on the basis of the location of the glomerulus and the path of the loop of Henle: the juxtamedullary nephrons and the cortical nephrons. Most nephrons are cortical in location, have short loops of Henle that extend into the superficial medulla, and have a relatively low capacity to reabsorb solute and water. Juxtamedullary nephrons are fewer in number but have longer loops of Henle that extend deep into the medulla. Consequently, these nephrons absorb larger amounts of salt and water, generate steep osmotic gradients, and produce highly concentrated urine. Regardless of the location of the nephron, the loop of Henle returns to the cortex to become a distal tubule. The distal tubule then continues on to form the collecting duct, the final common pathway for several nephrons draining into the renal papilla.
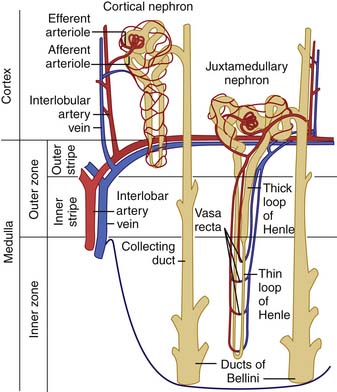
Figure 66–2 The nephron structure.
(From Guyton A: Formation of urine by the kidney: I. Renal blood flow, glomerular filtration, and their control. In Wonsiewicz M, editor: Textbook of medical physiology, ed 8, Philadelphia, 1991, WB Saunders.)
Nephron Development
Nephron development occurs through a complex, interactive series of processes that remains to be completely understood.1–3 Nephron development begins with the out-pouching of the ureteric epithelium, the ureteric bud. This precursor to the collecting duct encroaches on undifferentiated mesenchyme in the caudal retroperitoneal space and induces the development of an epithelial cell condensate, the precursor to the future glomerulus and tubule (Figure 66-3). Simultaneously, factors within the metanephric mesenchyme induce the ureteric bud to continue branching. The epithelial condensate forms a vesicle that convolutes progressively into a comma-shaped body and then an S-shaped body, signifying the development of the urinary space and early tubule segments. The terminal portion of the tubule is contributed by the ureteric bud derivatives and forms the collecting duct. The mechanisms by which the ureteric bud epithelial derivatives link to the corresponding mesenchymal derivatives in the distal nephron remain unknown. The glomerular capillary loops appear to form through the angiogenic processes of committed endothelial cells,5 and supporting mesangial cells develop from committed metanephric mesenchyme with myoblastic characteristics.12 Nephrogenesis in the human is essentially complete by the thirty-fourth week of gestation, but functional maturation continues into the second year of life.1–3
Glomerular Function
Filtration is the primary function of the glomerulus. For filtration to occur, there must be a gradient across the glomerular basement membrane favoring the movement of filtrate to a low-pressure area. There are generally four factors that determine the quantity of filtrate obtained across the glomerular basement membrane (Figure 66-4). First, hydrostatic pressure in the glomerular capillary drives filtration of fluid across the glomerular basement membrane. If the blood flow to the glomerulus decreases, the hydrostatic pressure also drops, which necessitates an increase in efferent vascular resistance to maintain glomerular perfusion pressure. Hormones, predominantly angiotensin II and prostaglandins, and renal sympathetic activity control afferent and efferent vascular tone to carefully regulate glomerular vascular resistance.
The last important factor in the determination of ultrafiltrate formation relates to the intrinsic properties of the glomerular basement membrane. Both the area of available membrane surface and the efficiency of the membrane to filter affect the generation of ultrafiltrate. Physiologically, the “size” of the glomerular basement membrane can be determined at the whole kidney level or at the glomerular level. At the whole kidney level, the number of nephrons receiving adequate blood supply determines glomerular basement membrane area available for filtration. For example, shunting of blood from the cortex into the medulla, as seen in persons with hepatorenal syndrome, effectively decreases the available glomerular basement membrane area by reducing the number of actively filtering nephrons. At the glomerular level, glomerular basement membrane area can be altered by mesangial cell function. In hypovolemic states, mesangial cell contraction is thought to decrease glomerular basement membrane area in response to hormonal mediators, resulting in decreased filtration and preservation of intravascular volume.13 The efficiency of basement membrane filtration also can be affected by disease states including immune complex deposition, fibrosis, or complement activation, which disrupt the integrity and efficiency of the membrane.
Finally, selectivity of the filtration barrier is determined by the ability of the basement membrane to permit some materials to pass into the urine while restricting others to stay in the blood compartment. Selectivity appears to be the result of both size discrimination of the glomerular basement membrane and the orientation of podocyte foot processes on the glomerular basement membrane. Disruption of the normal podocyte physiology results in nephrotic range proteinuria.14,15
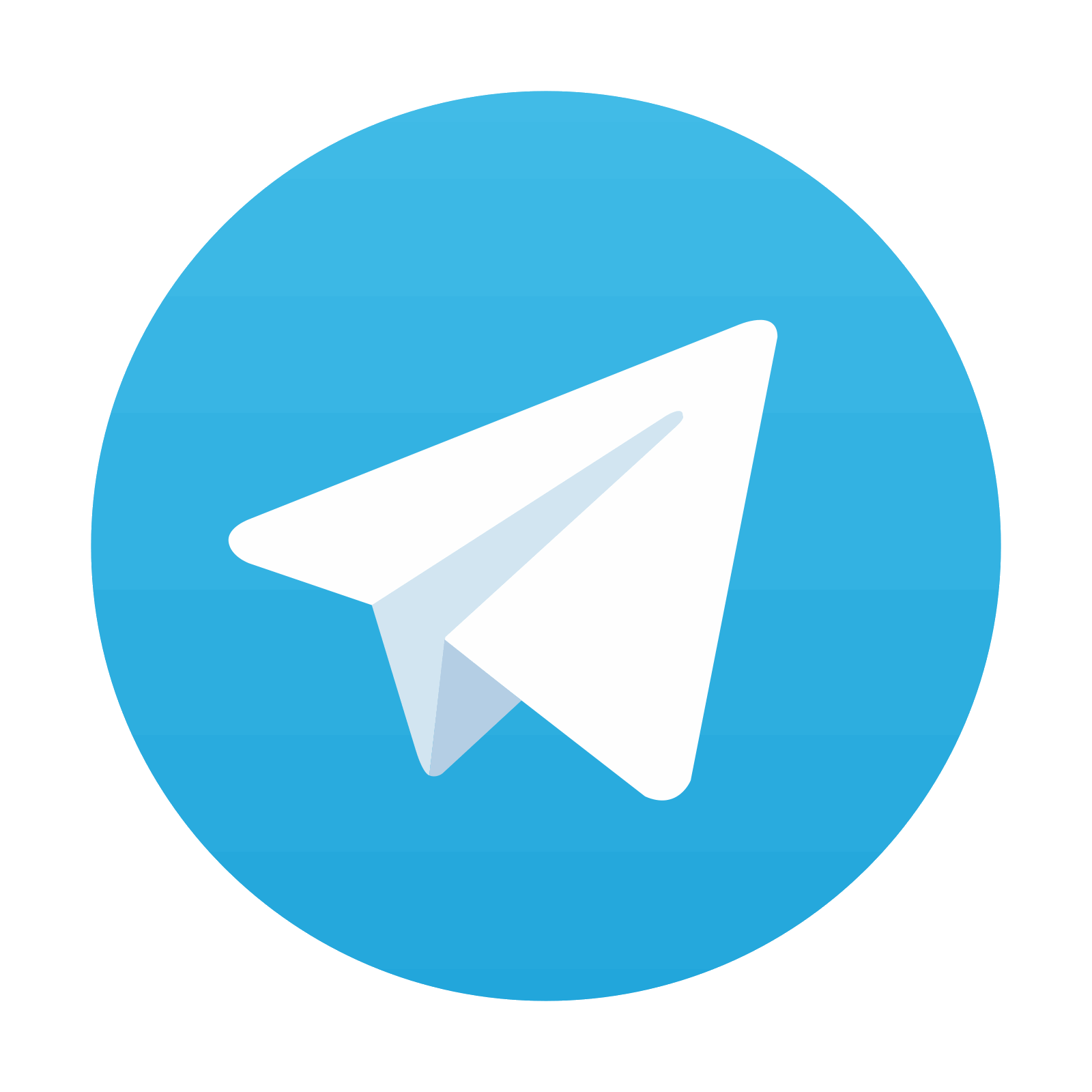
Stay updated, free articles. Join our Telegram channel
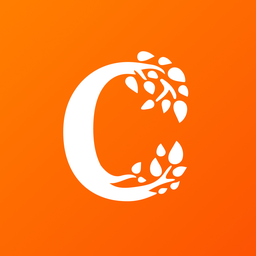
Full access? Get Clinical Tree
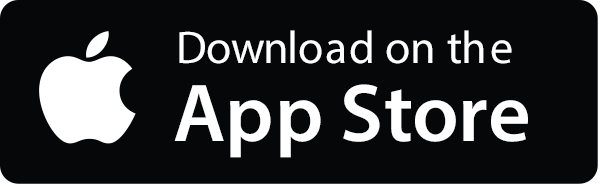
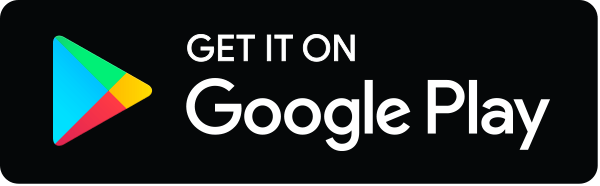