Chapter 37 Physiology of the Respiratory System
Physiology of the Respiratory System
Because of the increasing emphasis on molecular biology today, many physicians currently in training have received limited exposure to physiologic principles that form the basis of clinical medicine. However, a resurgence of interest in translational research has occurred recently with a reemphasis on molecular research and applied animal physiology before these principles are utilized in clinical research.1 In a recent editorial in the European Journal of Physiology, Rossier1a stated that research is refocusing itself to make a change from the past, where its mantra was “from function to the gene,” to the future, where the focus must be on the “gene to function.” The focus of this chapter will be to expose the reader to the important basic principles of respiratory physiology and to serve as a primer to other chapters in this book that utilize these principles.
Conducting Airways
Additional narrowing occurs during forced expiration, when there is dynamic airway compression caused by pleural and peribronchial pressures. This narrowing is counteracted by the intraluminal pressure and the tethering action of the surrounding lung. The luminal diameter of a branch is related to the number of alveoli at the end of that branch (axial and lateral pathways). Because the longer airways with more branches and more alveoli usually have a wider lumen that allows greater airflow, newly inspired air reaches all of the alveoli throughout both lungs at the same time and in approximately the same amount, that is, an even distribution of inspired air throughout all lobes in a given period of time. There are approximately 23 airway divisions to the level of the alveoli. The divisions include main bronchi, lobar bronchi, segmental bronchi (to designated bronchopulmonary segments), and so on to the smallest bronchioles, which do not have alveoli and are lined completely by bronchial epithelium. These are the terminal bronchioles. Although the base airway diameter decreases with branching, the overall or total cross-sectional diameter increases tremendously so that peripheral airway resistance decreases.
Model of the Respiratory System
A single balloon on a pipe is the simplest model, although this model has its deficiencies because the airway is more complex than a simple pipe. Also, it now appears that the alveolus is not simply a single balloon or group of balloons similar to a cluster of grapes. It is now known that the alveoli are not physically independent structures but are actually interconnected.2 An excellent review of the structure of the alveoli and the role of surfactant is offered by Gatto et al.3 However, to lay the ground work for our understanding of respiratory mechanics, we will consider the simple model of a balloon on a pipe.
where E is the elastance of the balloon, R is the resistance of the pipe, and is the flow through the opening. Using regression analysis, E and R can be calculated from P(t), V(t), and V′(t).
Each of these components will be discussed separately.
Elastic Properties of the Respiratory System
The respiratory system is composed of a collection of elastic structures. The response to a force applied to the elastic structure of the respiratory system is to resist deformation by producing an opposing force, known as elastic recoil, to return the structure to its relaxed state.4 In the respiratory system this opposing force produces a pressure known as the elastic recoil pressure (PEL). The force required to stretch an elastic structure depends on the volume at which the outward recoil of the chest wall balances the inward recoil known as the elastic equilibrium volume. The pressure of the elastic recoil or PEL divided by the lung volume (V) gives a measure of the elastic properties of respiratory system and is called elastance (E):
where Pao is pressure at the airway opening (usually atmospheric pressure), PA is the alveolar pressure, and Pfr is the pressure required to overcome frictional resistance. The pressure required to produce a unit of flow is known as flow resistance (R):
Traditionally it was thought that little energy was dissipated by the tissues of the respiratory system and that the majority of the force developed during breathing was required to move gas through the airways. The lung parenchyma is a complex system consisting of alveolar walls composed of collagen, elastin, and proteoglycan macromolecules; an air liquid interface of surfactant; and cells that have the capacity to act in a contractile fashion, called interstitial cells. The viscoelastic behavior of the pulmonary parenchyma could potentially explain this behavior. In addition, this action is difficult to study because it is unclear where the boundary of the airways end and parenchyma begins. Airway smooth muscle exists in the terminal bronchioles and alveolar ducts, and the behavior of these structures may well influence parenchymal mechanics.
The energy expended moving the tissue is called the tissue viscance or resistance, although it is a non-Newtonian resistance. In other words, the viscosity depends upon the force applied. When measured during inspiration, the tissue resistance increases with increasing lung volume, whereas airway resistance falls. Tissue resistance comprises approximately 65% of respiratory system resistance at FRC in mechanically ventilated animals and increases as much as 95% at higher lung volumes.5 The contribution of tissue resistance to respiratory system resistance in humans under the same circumstances is unknown.
Resistance is expressed as changes in pressure divided by changes in flow:
where P is the internal pressure, T is the tension in the wall of the structure, and r is the radius. When comparing two different alveoli with the same surface tension, the smaller the radius, the greater the pressure created by a given surface tension. Air will flow from high pressure (small alveoli) to lower pressure (larger alveoli). Thus smaller alveoli are more likely to collapse. The surface tension of the alveoli is affected by a substance produced in the alveoli called surfactant. Surfactant contains a mixture of lipids and proteins, is manufactured by alveolar type II cells, and exists as a monolayer on top of the alveolar subphase. Three surfactant-associated protein groups have been identified.6 Surfactant acts to lower surface tension at the alveolar air-liquid interface and thereby decreases elastic recoil of the lungs.7 Another action of surfactant is to reduce the development of pulmonary edema by diminishing one component of the pressure gradient driving transudation. In the lung there is a gradient between pulmonary capillary pressure and the interstitial pressure that surrounds the capillary. In most of the lung, the pulmonary capillary pressure is greater than the interstitial pressure; thus pulmonary edema would develop if not checked by the oncotic pressure of the plasma proteins. By reducing surface tension, the surfactant reduces the interstitial pressure and transcapillary gradient, but if there is a deficiency of surfactant and thus a rise in surface tension, pulmonary edema may develop.8 Also, surfactant has been described as an anti-wetting agent that helps to keep the lungs dry.9 Currently there is agreement on the fact that surfactant plays an essential role in alveolar mechanics, but its mechanism is debated. The aforementioned description outlines the classic discussion on the role of surfactant, but diverse opinions exist on its true role. Scapelli10 has described the role of the surfactant foam bubbles within the alveoli as “inner tubes.” In contrast, Hills proposes that surfactant coats the alveolar walls as a “biologic wax.”11,12 The clinical implications of a deficiency of surfactant has been described in a famous editorial by Lachman entitled “Open up the Lung and Keep the Lung Open.”13
Compliance and Elastance
Pulmonary compliance changes with growth and maturation depending upon the number of expanded air spaces, the size and geometry of the air spaces, the characteristics of the surface lining layer, the properties of the lung parenchyma. This shift is represented by changes in the shape of the volume-pressure curve. When these curves are corrected by expressing the volumes as a percentage of the maximal observed lung volume, they are more curved in infants than in older children (Figure 37-1).14 It is important to note that there may be boundaries for dynamic changes in alveolar size and shape during ventilation because of the tensile forces of the connective tissue and surface tension supporting the alveoli and alveolar ducts.
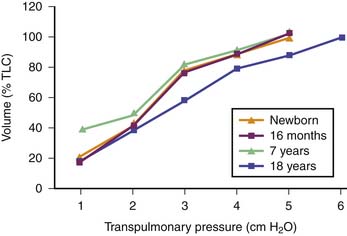
Figure 37–1 Deflation volume-pressure curves of the lung at different ages (obtained from studies on excised lungs).8 With increasing age up to young adulthood, the curves become straighter and, at a given lung volume, elastic recoil pressure is greater. The curve from elderly individuals resembles that from a 7-year-old respiratory system. TLC, Total lung capacity.
The developmental change in shape of the volume pressure curve represents the maturation of alveoli and hence differences in the elastin-collagen ratio with age.14 The lung volume (as a percent of maximal lung volume) at which airway closure occurs is higher in children younger than 7 years15 and is closer to their functional residual volume. Pressure-volume relationships are also more curvilinear in infants.16 Chest wall compliance is 50% greater in infants.
Elastance is therefore the reciprocal of compliance; thus stiff lungs have a high elastance.
Elastic Recoil of the Respiratory System
The pressure required to balance the elastic recoil of the lungs, chest wall, and respiratory system (elastic recoil pressure) may be determined by having a subject exhale in increments from total lung capacity (TLC) to residual volume. At each volume, the subject relaxes against a fixed obstruction with glottis open, and the pressure difference across the lung, chest wall, and entire respiratory system is recorded. Pressure volume curves are derived in this way for the respiratory system, and its components are shown in Figure 37-2.17 The static pressure-volume curves of the respiratory system, lung, and chest wall are different during inspiration and expiration. Thus lung volume at a given transpulmonary pressure is higher during deflation than during inflation. This phenomenon is called hysteresis. Hysteresis is the failure of a system to follow identical paths of response on application and withdrawal of a forcing agent, as occurs during inspiration and expiration. Hysteresis in the respiratory system depends on viscoelasticity, such as stress adaptation (i.e., rate-dependent phenomenon), and on plasticity (i.e., a rate-independent phenomenon). In the lungs, hysteresis is due primarily to surface properties and alveolar recruitment-derecruitment. In comparison, the chest wall hysteresis is related to the action of both muscles and ligaments because both skeletal muscles and elastic fibers exhibit hysteresis. Hysteresis is negligible when volume changes are minimal, such as during quiet breathing. This phenomenon is important because the area of the hysteresis loop represents energy lost from the system.
Flow Resistance of the Respiratory System
Airways resistance (Raw) is the sum of the peripheral airways resistance (peripheral intrathoracic airways <2 mm diameter; Rawp), the central airways resistance (large intrathoracic airways >2 mm diameter; Rawc), and the extrathoracic airways resistance (especially glottis; Rext). In healthy people, Rext accounts for 50% of the total Raw and Rawp for about 15%. Rawp and Rawc are influenced by lung volume. Higher lung volumes give higher Pel and therefore increase airway diameter. With increasing volumes during inspiration, the increased Pel is counteracted by Ppl, resulting in increased radial distending force. This distending force is the transmural pressure and is the difference between pressure in (Pin) and pressure outside (Pout) the airway.
In older children, Rcw and RL represent only 10% to 20% of Rrs,18 but in newborns, RL could be higher.19
where Pao, Palv, Ppl, and Pbs represent the pressure at the airways opening, alveolar pressure, pleural pressure, and pressure at the body surface, respectively. The resistance of the lung parenchyma may be derived by subtracting airway from total lung resistance.
The relationship between the flow rate and the airway pressure gradient is nonlinear because of the relative contribution of the various components of the respiratory system to the total pressure required to overcome the viscous forces and its dependence upon volume, volume history, and flow. The viscous forces increase disproportionately as the flow rate increases and as airway resistance increases. In contrast, the resistance of the chest wall and lung parenchyma remains constant over a wide range of flow rates.20 During quiet breathing by mouth, airway resistance accounts for greater than 50% of the total respiratory system resistance.21 However, as flow rate increases, the contribution of the airways to total resistance progressively increases.
Changing patterns of airflow result in the nonlinear flow-resistance characteristic of the airways. Subsequently, as the flow rate to the airway increases, airflow becomes progressively more turbulent. The more turbulent the flow, the greater the pressure required to overcome the viscous forces. Turbulence occurs at lower flow rates in the upper airway compared with the lower (intrathoracic) airways because of the tortuous geometry of the upper (extrathoracic) airway and the narrow glottic aperture. Therefore, the upper airway is responsible for most of the increase in airway resistance with an increase in flow rate. Studies have shown the resistance of the lower airways to be nearly constant up to flow rates of 2L per second.20 For patients who are breathing quietly by mouth, total airway resistance is divided almost equally between the upper and lower airways. As their effort increases, flow rate increases, and the ratio of upper to lower airway resistance progressively increases as previously described.
Depending on whether laminar or turbulent flow predominates, resistance to airflow varies inversely with either the fourth or the fifth power of airway radius.22 Therefore major changes in airway resistance are caused by factors that affect airway diameter.23 During spontaneous lung inflation, airway diameter increases as airway resistance decreases. This change is produced by two mechanisms. First, as lung volume increases, the increasing elastic recoil of the pulmonary parenchyma provides a tethering effect that dilates the intrapulmonary airways. Second, extrapulmonary and large intrapulmonary airways are surrounded by pleural pressure, which becomes increasingly negative during inspiration. This phenomenon leads to an increasing pressure gradient across the airway wall and therefore to an increasing diameter. The change in airway resistance with lung volume is curvilinear and is illustrated in Figure 37-3.23 When the reciprocal of airway resistance, airway conductance (GAW) is plotted against lung volume, this relationship is nearly linear.
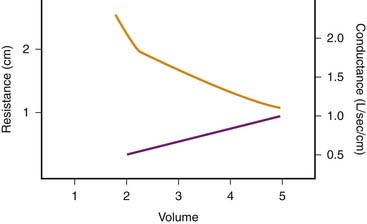
Figure 37–3 Relationship between lung volume and airway resistance (solid line) and conductance (dashed line).
(Modified from Briscoe WA, Dubois AB: The relationship between airway resistance, airway conductance, and lung volume in subjects of different age and body size, J Clin Invest 37:1280, 1958.)
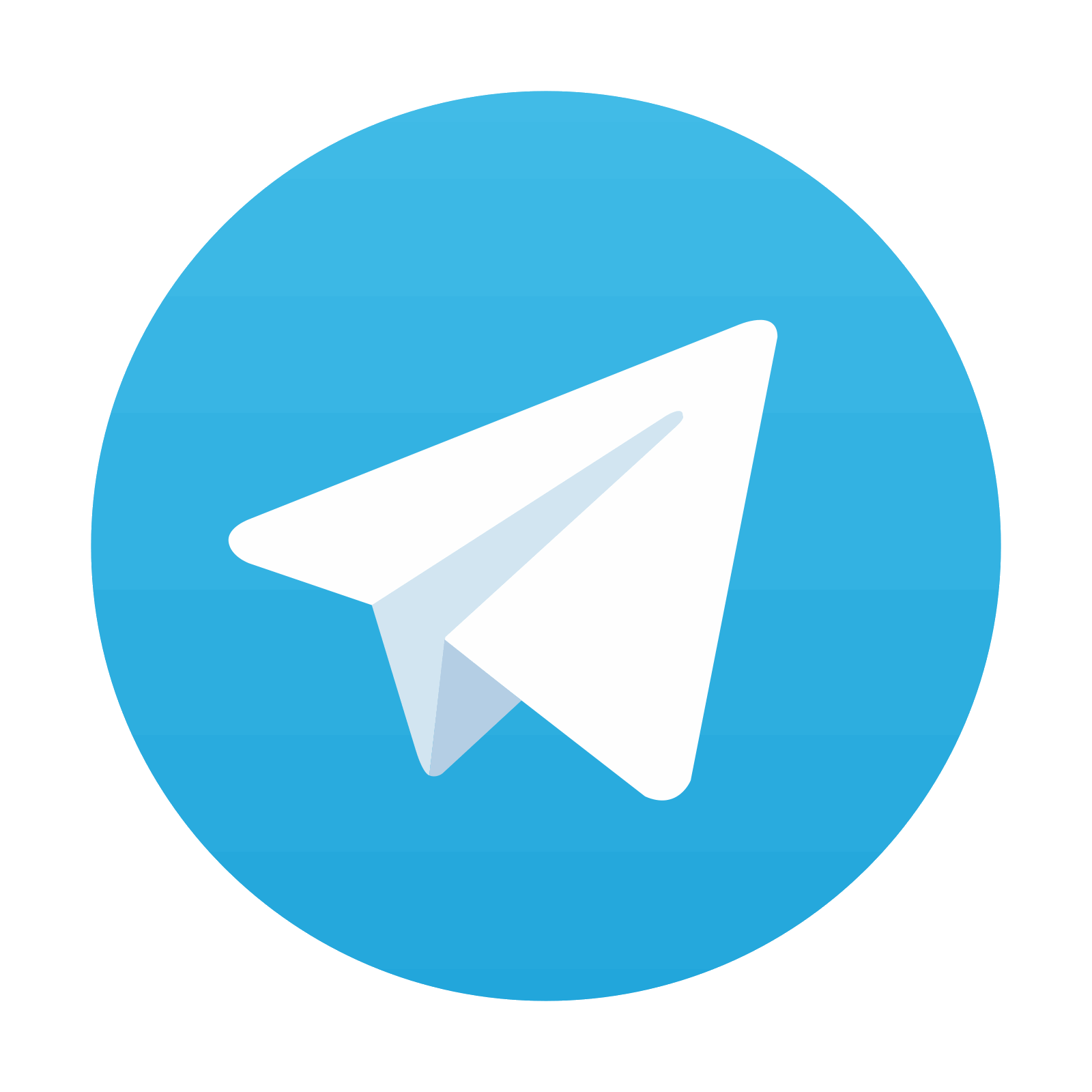
Stay updated, free articles. Join our Telegram channel
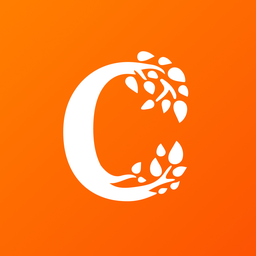
Full access? Get Clinical Tree
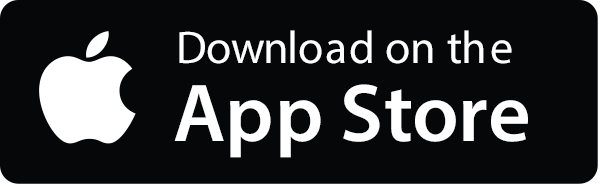
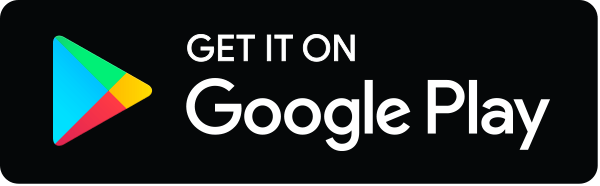