Chapter 33 Physiologic Foundations of Cardiopulmonary Resuscitation
With the development of basic cardiopulmonary resuscitation (CPR) in the early 1960s, skilled resuscitation teams both in and out of the hospital were formed. The development of CPR saved lives; previously every victim of cardiac arrest had died. Soon thereafter, successful resuscitation of patients by basic life support measures, defibrillation, and medications became common even as long as 5 hours after commencement of CPR. Data show that the success of CPR depends on many factors. Rapid institution of basic life support measures (i.e., bystander CPR for sudden out-of-hospital cardiac arrest and immediate electrical countershock for ventricular fibrillation (VF) improve the chances of survival for patients experiencing sudden out-of-hospital cardiac arrest.1 These measures led to the growing deployment of automatic external defibrillators (AEDs) in public places. Although immediate defibrillation currently is the standard of care, accumulating evidence indicates that basic life support and other measures directed at restoring energy substrates to the myocardium before countershock in patients with prolonged VF may further improve outcome.2–4
Other preexisting factors that play a role in successful resuscitation include the patient’s age, prior medical condition, presenting cardiac rhythm, and the etiology of cardiac arrest. In 2008, a multiinstitutional prospective study was published that examined these preexisting factors and further described in two additional studies the clinical characteristics, hospital course, and outcomes of a cohort of children after in-hospital or out-of-hospital arrest. Besides demonstrating differences in clinical characteristics, these studies offered future considerations for the care of children who had experienced cardiac arrest and postresuscitative care, including hypothermia.5,6 The low resuscitation rate in children, even when the patient does not have preexisting disease, probably results from the high incidence of asystole as the presenting rhythm. Asystole is the most common presenting rhythm in both in-hospital and out-of-hospital arrests, and is noted in 55% to 70% of victims.7–11 Bradycardia and pulseless electrical activity (PEA) are other common rhythms. The high incidence of asystole in children who experience cardiac arrest can be explained by systemic disturbances such as hypoxia, acidosis, sepsis, and hypovolemia that commonly precede the arrest. Although ventricular arrhythmias usually are reported to be infrequent (range, 1.3% to 3.8%),12 out-of-hospital series report VF in 10% to 19% of victims younger than 20 years.13 These series, along with the observation that the frequency of witnessed arrest is much lower than in adults,14 suggests that ventricular rhythms may be more common than usually estimated and that delay in resuscitation results in progression of nonperfusing rhythms to asystole. Increasing availability of AEDs may be contributing to the increased recognition of ventricular arrhythmias in out-of-hospital pediatric cardiac arrest. In specialized cardiac intensive care units (ICUs), ventricular arrhythmias account for as many as 30% of the arrests.15
In their original work on CPR, Kouwenhoven et al.16 proposed that blood flow during closed-chest compressions resulted from squeezing of the heart between the sternum and vertebral column, now termed the cardiac blood flow mechanism. In fact, the precise mechanism by which forward circulatory flow is generated during closed-chest cardiac massage has major implications for current approaches to CPR. Other methods, such as vest CPR, simultaneous compression ventilation CPR (SCV-CPR), active compression-decompression CPR (ACD-CPR) both without and with an impedance threshold valve (ITV), and interposed abdominal compressions with CPR (IAC-CPR), take into account advances in our understanding of the mechanism of blood flow during resuscitation.
The pharmacology of resuscitation remains controversial, and these controversies have led to major changes in the guidelines for CPR. Use of sodium bicarbonate, calcium chloride, and glucose remains unresolved at this time. The role of epinephrine and especially high-dose epinephrine has been readdressed because of concerns over postresuscitation deleterious effects on myocardial performance and poor outcomes. Evidence favoring a role for vasopressin, with a relatively pure vasoconstrictor effect, is accumulating. The role of lidocaine as the antiarrhythmic of choice for ventricular ectopy has been questioned as new data on the efficacy of amiodarone in persons in cardiac arrest have been generated. Research is ongoing into alternative vasoconstrictors17 and the use of “pharmacologic cocktails” that may include β-blockers, antiarrhythmic agents, antioxidants, nitroglycerin,1,18,19 and a vasoconstrictor in attempts to improve the resuscitation outcome and postresuscitation cardiac function.
Mechanisms of Blood Flow
Cardiac Versus Thoracic Pump Mechanism
Numerous clinical observations have conflicted with the cardiac pump hypothesis of blood flow. In 1964, Mackenzie et al.20 found that closed-chest CPR produced similar elevations in arterial and venous intravascular pressures, the result of a generalized increase in intrathoracic pressure. In 1976, Criley et al.21 made the dramatic observation that several patients in whom VF developed during cardiac catheterization produced enough blood flow to maintain consciousness by repetitive coughing (Figure 33-1). The production of blood flow by increasing thoracic pressure without direct cardiac compression describes the thoracic pump mechanism of blood flow during CPR.
Echocardiographic studies show that, unlike normal cardiac activity or during open-chest CPR, during closed-chest CPR in both dogs22 and humans,23 the atrioventricular valves are open during blood ejection and aortic diameter decreases rather than increases during blood ejection. These findings during closed-chest CPR support the thoracic pump theory and argue that the heart is a passive conduit for blood flow (Figure 33-2).24
Initial measurements of hemodynamic data during chest compression for CPR found the generation of almost equal pressures in the left ventricle, aorta, right atrium, pulmonary artery, and esophagus (Figure 33-3).25 The finding that all intrathoracic vascular pressures are equal implies that suprathoracic arterial pressures must be higher than suprathoracic venous pressures. The unequal transmission of intrathoracic pressure to the suprathoracic vasculature establishes the gradient necessary for blood flow. The transmission of intrathoracic pressure to the suprathoracic veins may be modulated by venous valves. The presence of these jugular venous valves has been demonstrated in animals26 and humans27,28 undergoing CPR. An ultrasonography study of healthy children confirmed the presence of these valves in 84% of 239 jugular veins studied. The valves were bilateral in 74% of children.29 Transmission of intrathoracic pressure to the intracranial vault during CPR indicates that any such valve function is partial. Pathologic studies have also identified valves in the subclavian vein in the large majority of cadavers studied (87%). The absence of these valves in some patients is postulated to lead to failure of closed-chest CPR.30
Subsequent hemodynamic and echocardiographic studies found different results. Deshmukh et al.31 demonstrated in a porcine model that mitral valve function persisted throughout resuscitation in 17 of 22 animals and that in successfully resuscitated animals, maximal aortic pressure exceeded that in the right atrium throughout the resuscitation. In another porcine model of resuscitation, Hackl et al.32 manipulated the compressive force and depth of resuscitation by using a mechanical resuscitator. The frequency of mitral valve closure during compressive systole was directly proportional to the force and depth of chest compression. When the depth of compression reached 25% of the anteroposterior diameter, valve closure occurred in 95% of cycles. They concluded that the mechanism of blood flow was dependent on the force and depth of compression. In a study of CPR using transesophageal Doppler echocardiography in adults, Porter et al.33 demonstrated mitral valve closure in compressive systole in the majority of patients (12 of 17) but not all patients. Peak mitral flow occurred in diastole and was significantly higher in the group with mitral valve closure. Peak mitral flow occurred during compressive systole in those without valve closure. Left ventricular fractional shortening correlated with change in anteroposterior chest wall diameter and not mitral valve flow. These authors concluded that nonuniform increased intrathoracic pressure plays a role in determining whether valve closure occurs during chest compressions. As noted, a decrease in aortic dimension during CPR has been demonstrated by echocardiography and taken as evidence for the thoracic pump mechanism of blood flow. Hwang et al.34 readdressed this issue using transesophageal echocardiography. They studied the aortic dimension of the proximal and distal thoracic aorta and noted a decrease in the aortic dimension in the distal aorta directly inferior to the zone of direct compression and an increase in the dimension of the proximal aorta. They also noted mitral valve closure in all subjects and a decrease in left ventricular (LV) volume of almost 50% at end compression. These findings were believed to be most consistent with the cardiac pump mechanism of blood flow.35 Kim et al.36 also used transesophageal echocardiography to explore the role of the LV during nontraumatic arrests. They noted that during the compression phase of CPR, there was anterograde flow from the ventricle to the aorta, as well as retrograde flow toward the mitral valve. The mitral valve remained closed during compression and open during relaxation, while the aortic valve remained open during compression and closed during relaxation, which they concluded to be consistent with the cardiac pump mechanism.
The cardiac pump mechanism appears to predominate during closed-chest CPR in specific clinical situations. As noted, increasing the applied force during chest compressions increases the likelihood of direct cardiac compression.32,36 A smaller chest size may allow for more direct cardiac compression. Adult dogs with small chests have better hemodynamics during closed-chest CPR than do dogs with large chests. Because the infant chest is smaller and more compliant than the adult chest, direct compression of the heart during CPR is more likely to occur. Blood flow during closed-chest CPR in a piglet model of cardiac arrest is higher than that achieved in adult models.37 In contrast to adult animals, increasing intrathoracic pressure by SCV-CPR does not augment vascular pressure or regional organ blood flow during CPR in piglets.38 The failure of SCV-CPR to increase blood flow in the infant implies that direct compression occurs with conventional CPR and that additional intrathoracic pressure is of no benefit.
Rate and Duty Cycle
In 2010 the American Heart Association (AHA) recommended a rate of chest compressions of at least 100 per minute.39 At faster rates, blood flow is enhanced whether the thoracic pump mechanism or the cardiac pump mechanism is invoked. Duty cycle is defined as the ratio of the duration of the compression phase to the entire compression-relaxation cycle expressed as a percent. For example, at a rate of 30 compressions/min, a 1.2-second compression time produces a 60% duty cycle. If blood flow is generated by direct cardiac compression, then the stroke volume is determined primarily by the force of compression. Prolonging the compression (increasing the duty cycle) beyond the time necessary for full ventricular ejection should have no additional effect on stroke volume. Increasing the rate of compressions should increase cardiac output because a fixed, relatively small volume of blood is ejected with each cardiac compression. In contrast, if blood flow is produced by the thoracic pump mechanism, the volume of blood to be ejected comes from a large reservoir of blood contained within the capacitance vessels in the chest. With the thoracic pump mechanism, flow is enhanced by increasing either the force of compression or the duty cycle but is not affected by changes in compression rate over a wide range of rates.27 Additionally, the “push hard, push fast” recommendation is based on the maintenance of a higher compression rate with a higher force of compression. Allowing total recoil of the chest allows for full blood return during the relaxation phase of the cycle.40
Mathematical models of the cardiovascular system confirm that blood flow is determined by both the applied force and the compression duration with the thoracic pump mechanism.41 A mathematical model equating CPR to a circuit, constructed by Babbs,42 determined that while hemodynamics did not vary with compression rate, total flow and coronary flow were greatest when compression time equaled 30% of cycle time.
Results of several studies in dogs demonstrated a benefit of a compression rate of 120 per minute compared with slower rates during conventional CPR.43,44 In studies of piglets,45 puppies,46 and humans,27,47 no differences were found comparing different rates of compression during conventional CPR. In a study of piglet CPR, duty cycle was the major determinant of cerebral perfusion pressure. The duty cycle at which venous return became limited varied with age. A longer duty cycle was more effective in younger piglets.45
Chest Geometry
The change in cross-sectional area of the chest during anterior to posterior delivered compressions is related to its shape (Figure 33-4).48 The ratio of the chest anteroposterior diameter to the lateral diameter is referred to as the thoracic index. A keel-shaped chest, as seen in an adult dog, has a greater anteroposterior diameter and thus a thoracic index greater than 1. A flat chest, as in a thin human, has a greater lateral diameter and thus a thoracic index less than 1. A circular chest has a thoracic index equal to 1. A circle has a larger cross-sectional area than either of these elliptical chests. As an anteroposterior compression flattens a circle, the cross-sectional area decreases and compresses its contents. In contrast, as an anteroposterior compression is applied to the keel-shaped chest, the cross-sectional area increases as a circular shape is approached. The cross-sectional area of the keel-shaped chest does not decrease until the chest compression continues past the circular shape to flatten the chest. This implies a threshold past which the compression must proceed before intrathoracic contents are decreased and squeezed.48 Thus the rounder, flatter chests of small dogs and pigs may require less chest displacement than the keel-shaped chests of adult dogs to generate thoracic ejection of blood. This dynamic has been demonstrated in small dogs having round chests compared with adult dogs having keel-shaped chests.49
As humans age, the cartilage of the rib cage calcifies and chest wall compliance decreases. Older patients may require greater compression force to generate the same sternal displacement. A 3-month-old piglet requires a much greater compression force for anteroposterior displacement than its 1-month-old counterpart.48 Direct cardiac compression is more likely to occur in the more compliant chest of younger animals. Cerebral and myocardial blood flow during closed-chest CPR was much higher in infant piglets than in adults50 (Figures 33-5 and 33-6). This finding supports the cardiac pump mechanism of blood flow in infants because the level of organ blood flow achieved during closed-chest CPR in piglets approaches the level achieved during open-chest cardiac massage in adults.
Marked deformation of the chest can occur during prolonged CPR and may alter the effectiveness of CPR (Figure 33-7).50 Over time, the chest assumes a flatter shape, producing a larger percent decrease in cross-sectional area at the same absolute chest displacement. Progressive deformation may be beneficial if it leads to more direct cardiac compression. Unfortunately, too much deformation may decrease the recoil of the chest wall during the relaxation phase, leading to decreased cardiac filling. A progressive decrease in the effectiveness of chest compressions to produce blood flow is seen in piglets receiving conventional CPR.50 Permanent deformation of the chest in this model approaches 30% of the original anteroposterior diameter. Attempting to limit deformation by increasing intrathoracic pressure from within during CPR with SCV-CPR was ineffective.51 Using a thoracic vest to limit deformation when performing CPR greatly decreased the permanent chest deformation (3% vs. 30%) but did not attenuate the deterioration of vital organ blood flow with time.52
The characteristics of chest geometry of animals may relate to that in humans. Body weight, surface area, chest circumference, and diameter did not correlate with the magnitude of aortic pressure produced during CPR in a study of nine adults already declared dead.52 A direct comparison of adult and pediatric human CPR has not been performed. The higher intravascular pressures and organ blood flow during CPR in infants compared with adults may result from more effective transmission of the force of chest compression because of the higher compliance and greater deformability of the infant chest.
Effects of Cardiopulmonary Resuscitation on Intracranial Pressure
When chest compressions are applied, the increase in intrathoracic pressure is transmitted through the venous system of the head and neck to the intracranial vault, resulting in an increased intracranial pressure (ICP). Pressure is transmitted via the paravertebral veins and the cerebrospinal fluid during CPR in dogs.53 Large swings in ICP corresponding to chest compressions occur in children undergoing CPR (Figure 33-8).54 This transmission of intrathoracic pressure to the intracranial contents accounts for the low cerebral perfusion pressure by increasing the downstream pressure and cerebral blood flow during closed-chest CPR.
The relationship of ICP to intrathoracic pressure during CPR is linear. In dogs receiving conventional CPR, ICP increased by one third of the rise of intrathoracic pressure in a range from 10 to 90 mm Hg.53 However, some modes of CPR change the intrathoracic to ICP relationship. In dogs, abdominal binding increases the transmission of pressure to the intracranial space to one half of the rise of intrathoracic pressure.55 SCV-CPR, a mode of CPR designed to generate higher intrathoracic pressure, is similar to conventional CPR in its transmission of pressure to the cranium. Open-chest CPR decreases the transmission of pressure and improves cerebral perfusion pressure compared with conventional CPR. Thus increasing intrathoracic pressure may decrease cerebral blood flow because of the increase in downstream pressure, the ICP.
In this regard, ACD-CPR and ACD-ITV-CPR may have an advantage over conventional CPR. These techniques are designed to reduce intrathoracic pressure. Lindner et al.56 showed in a porcine model that cerebral perfusion is increased with ACD-ITV-CPR compared with standard CPR. Using an adult porcine model of hypothermic VF arrest, the same group demonstrated by microdialysis techniques improved lactate/pyruvate ratios and reduced glucose accumulation in the ACD-ITV group compared with standard CPR.57 In a pediatric porcine model of resuscitation, Voelckel et al.58 found that ACD-CPR with ITV provided superior cerebral blood flow compared with standard CPR.
Newer Cardiopulmonary Resuscitation Techniques
Simultaneous Compression Ventilation Cardiopulmonary Resuscitation
Experimental studies have shown that SCV-CPR increases carotid blood flow compared with conventional CPR alone.59 Subsequent studies confirmed physiologic advantages of SCV-CPR in canine models.25 However, in infant piglets51 and small dogs,49 SCV-CPR offered no advantage over conventional CPR. In these small animals, the compliance and geometry of the chest may allow more direct cardiac compression. Thus higher intrathoracic pressure may be achieved with conventional CPR alone.45,50 Coronary perfusion pressure (CPP) was either only minimally increased or even decreased in humans during SCV-CPR compared with conventional CPR. Survival was significantly worse in both animals60 and humans61 who received SCV-CPR compared with conventional CPR. No study has shown an increased survival rate with this technique of CPR.
Interposed Abdominal Compression Cardiopulmonary Resuscitation
IAC-CPR is the delivery of an abdominal compression during the relaxation phase of chest compression. An extensive review by Babbs62 has been published. IAC-CPR may augment conventional CPR in several ways. First, IAC-CPR may return venous blood to the chest during chest relaxation.63,64 Second, IAC-CPR increases intrathoracic pressure and augments the duty cycle of chest compression.63,65 Third, IAC-CPR may compress the aorta and return blood retrograde to the carotid or coronary arteries.64 IAC-CPR is an attractive alternative to some of the newer techniques of CPR because it requires no additional equipment for implementation; however, it does require training and manpower.
In animal experiments, cardiac output and cerebral and coronary blood flow were improved when comparing IAC-CPR with conventional CPR,66 but not in an infant model.67 Initial human studies also demonstrated an increase in aortic pressure and CPP during IAC-CPR compared with conventional CPR.68 Four randomized controlled trials have compared IAC-CPR with standard CPR. The first trial reported in 1985 by Mateer et al.69 was the largest and included 291 patients. IAC-CPR was applied in the field by paramedics until ambulance transport. No differences in mortality were found. The later trials involved a total of 279 hospitalized patients.70,71,269 The results from these trials are more positive, and a meta-analysis of these studies found an increased likelihood of return of spontaneous circulation (ROSC) and intact survival to discharge with IAC-CPR versus standard CPR.72 Although no intraabdominal trauma was detected in any of the 426 patients in these trials, one pediatric case report demonstrated direct pancreatic injury. Alternative techniques for abdominal hand position were studied in adult swine.73,74 A stacked hand position similar to the usual position for chest compression over the abdominal aorta was compared with a diffuse hand position in which the hands were placed on the abdomen separately. This study demonstrated a significant increase in aortic diastolic pressure compared with standard CPR. However, CPP was not augmented because the right atrial diastolic pressure was also elevated. Stacked hand position was found to produce a CPP equivalent to standard CPR. Diffuse hand position, however, was associated with decreased CPP, so if the technique is applied, this hand position should be avoided. Application of IAC-CPR is limited by the need for training and for the additional manpower. Although it has not been studied in a pediatric group, with skilled personnel available, IAC-CPR should be considered for use with inpatient arrests.
Active Compression-Decompression Cardiopulmonary Resuscitation and Impedance Threshold Valve Interposition
ACD-CPR uses a negative pressure “pull” on the thorax during the release phase of chest compression using a hand-held suction device (Figure 33-9).75 This technique improves vascular pressures and minute ventilation during CPR in animals77,78 and humans.75,79 The mechanism of benefit of this technique is attributed to enhancement of venous return by the negative intrathoracic pressure generated during the decompression phase; in addition, it reverses the chest wall deformation that accompanies standard CPR.80 Preliminary results in adults were promising,77,81,82 and a large multiinstitutional study of ACD-CPR completed in Europe found that ACD-CPR was superior to standard CPR. In this study, a total of 750 patients were randomly assigned to receive standard CPR or ACD-CPR. In the experimental group, 5% survived to 1 year (12 patients with intact neurologic status), versus 2% (three patients with intact neurologic status) in the standard group.83 However, a number of other trials have not shown a difference between standard CPR and ACD-CPR. A Cochrane Database Systematic Review concluded there was no consistent benefit from use of this technique.84 The effectiveness of ACD-CPR appears to be relatively site specific. Explanations for this variability have focused on the effectiveness of training for providers and intersite variation of on-scene advanced life support techniques.80 Use of ACD-CPR requires significantly more physical effort than conventional CPR, and this requirement may have influenced outcome.85 No device is currently cleared for clinical use in the United States at this time.
Use of an inspiratory threshold valve has been evaluated in attempts to improve the outcome with ACD-CPR.83,86 This technique involves the use of a valve placed between the ventilating bag and the airway, which is designed to close when the tracheal pressure falls below atmospheric pressure, enhancing the development of negative intrathoracic pressure during ACD-CPR (Figures 33-10 and 33-11). Animal studies,87,88 including a young porcine model,58 showed improved organ perfusion, and brain microdialysis studies demonstrated decreased lactate accumulation and improved glucose utilization.57 In a small series of patients, diastolic pressure was raised along with CPP and end-tidal CO2 (EtCO2) release.89 These studies led to an inclusion of the technique as an acceptable alternative to standard CPR in the 2000 AHA guidelines and subsequent revised guidelines.90 Plaisance et al.83 reported on a series of 400 patients randomly assigned to ACD-CPR with ITV or sham ITV. Survival at 24 hours was significantly improved. There was a nonsignificant trend toward improved neurologic survival, with 6 of 10 discharged patients having intact survival compared with 1 of 8 discharged survivors in the sham ITV group. In two randomized control studies by Wolcke et al. and Plaisance et al. of 610 adults in cardiac arrest in the out of hospital setting, use of ACD-CPR plus the ITD was associated with improved ROSC and 24-hour survival rates when compared with CPR alone.91 The addition of the ITD was associated with improved hemodynamics during standard CPR in one clinical study.90 The ultimate role of this technique, which requires specialized equipment and significant resuscitator training, remains to be determined.83,92
Vest Cardiopulmonary Resuscitation
Vest CPR uses an inflatable bladder resembling a blood pressure cuff that is wrapped circumferentially around the chest and inflated phasically to increase intrathoracic pressure. Because chest dimensions are changed minimally, direct cardiac compression is unlikely. In addition, the even distribution of the force of compression over the entire chest wall decreases the likelihood of trauma to the skeletal chest wall and its thoracic contents.
Improvement of cerebral and myocardial blood flows93,94 and survival95,96 with vest CPR compared with conventional CPR was seen in dogs. In piglets, a 3% permanent chest deformation was seen after 50 minutes of vest CPR,52 compared with an almost 30% deformation produced during an equivalent period of conventional CPR.50 In a human study, vest CPR increased aortic systolic pressure but had little effect on aortic diastolic pressure compared with conventional CPR.97 Despite its late application, vest CPR improved the hemodynamics and the rate of ROSC in adult patients in another study.98 Evidence from a case control study of 162 adults documented improvement in survival to the emergency department when vest CPR was administered by adequately trained personnel to patients in cardiac arrest in the out-of-hospital setting.99 The lack of metallic parts has allowed vest CPR to be used experimentally during nuclear magnetic resonance spectroscopy to study brain intracellular pH.100 The vest also has been used as an external cardiac assist device in nonarrested dogs with heart failure.101 Clinically, the use of vest CPR depends on sophisticated equipment and remains experimental at this time.
Abdominal Binding
Abdominal binders and military antishock trousers have been used to augment closed-chest CPR. Both methods apply continuous compression circumferentially below the diaphragm. Three mechanisms have been proposed for augmentation of CPR by these binders. First, binding the abdomen decreases the compliance of the diaphragm and raises intrathoracic pressure. Second, blood may be moved out of the intrathoracic structures to increase circulating blood volume. Third, applying pressure to the subdiaphragmatic vasculature and increasing its resistance may increase suprathoracic blood flow. These effects increase aortic pressure and carotid blood flow in both animals25 and humans.102 Unfortunately, as aortic pressure increases, the downstream component of CPP, namely, right atrial pressure, increases to an even greater extent, resulting in decreased CPP and myocardial blood flow.22 These techniques also lower the cerebral perfusion pressure by enhanced transmission of intrathoracic pressure to the intracranial vault, which raises ICP (the downstream component of cerebral perfusion pressure). Clinical studies have failed to show an increased survival when an abdominal binder or military antishock trouser suit was used to augment CPR.
Open-Chest Cardiopulmonary Resuscitation
Use of open-chest cardiac massage has generally been replaced by closed-chest CPR. Compared with closed-chest CPR, open-chest CPR generates higher cardiac output and vital organ blood flow. During open-chest CPR there is less elevation of intrathoracic, right atrial, and intracranial pressure, resulting in higher coronary and cerebral perfusion pressure and higher myocardial and cerebral blood flow.103
Open-chest CPR is not a technique that can be applied by most health care personnel. It can be used in the operating room, ICU, or emergency department equipped with the necessary surgical and technical equipment and personnel. It is easily used in the operating room or ICU after cardiac surgery when the open chest can be easily accessed. Open-chest CPR is indicated for cardiac arrest resulting from cardiac tamponade, hypothermia, critical aortic stenosis, and ruptured aortic aneurysm. Other indications include cardiac arrest resulting from penetrating or crushed chest wall abnormalities that make closed-chest CPR impossible or ineffective.40 Open-chest CPR is indicated for select patients when closed-chest CPR has failed, although exactly which patients should receive this method of resuscitation under this condition is controversial. When initiated early after failure of closed-chest CPR, open-chest CPR may improve outcome.104 When performed after 15 minutes of closed-chest CPR, open-chest CPR significantly improves CPP and the rate of successful resuscitation.105
Cardiopulmonary Bypass
Because of the low rate of survival after prolonged CPR, more aggressive methods have been suggested to improve its success: cardiopulmonary bypass (CPB) and extracorporeal membrane oxygenation CPR.39
CPB is one of the most effective ways to restore circulation after cardiac arrest. Animal studies show that CPB increases survival at 72 hours, increases recovery of consciousness, and preserves the myocardium better than does conventional CPR.106 In dogs, CPB resulted in better neurologic outcome than conventional CPR after a 4-minute ischemic period; however, neurologic outcome was dismal in both groups when the ischemic period lasted 12 minutes.106 Some 90% of dogs survived 24 hours after 15 to 20 minutes of cardiac arrest, but only 10% survived when the arrest time was prolonged to 30 minutes when CPB was used for stabilization during defibrillation.108 CPB decreased myocardial infarct size in a model involving coronary artery occlusion compared with conventional CPR.109 In all animal models, CPB improves the success of resuscitation compared with conventional CPR.
Human experience with CPB for cardiac arrest outside the operating room is growing. Morris et al.110 reviewed these data. Accumulated experience now includes seven pediatric series reporting on 127 cases and 10 adult series reporting on 331 cases. Overall survival is excellent compared with conventional CPR despite long arrest times. In both groups, survival to decannulation is approximately 60% despite average precannulation CPR times, where reported, ranging from 16 to 60 minutes for children and from 20 to 80 minutes for adults. Long-term survival is remarkable, with 47% of children and 31% of adults achieving long-term survival. In the largest series of children reported by Morris et al.,110 between 1995 and 2002, 64 children underwent 66 extracorporeal membrane oxygenation (ECMO) runs initiated during active resuscitation with chest compressions or internal cardiac massage. Of these patients, 33 (50%) were decannulated and survived for more than 24 hours, 21 (33%) survived to hospital discharge, and 16 (26%) reportedly had no major changes in neurologic outcome. The average duration of CPR before cannulation in the survivors was 50 minutes. Of the six surviving children who required more than 60 minutes of CPR before ECMO, three had no apparent change in neurologic status. During the same period, 73 children underwent standard CPR; 10 received CPR for more than 30 minutes, with no survivors. Duncan et al.111 reported a series of 18 pediatric cardiac surgical patients at the Boston Children’s Hospital who received ECMO during active chest compressions. Of the first seven patients, only 29% survived. This led to the development of a rapid ECMO deployment strategy in which an ECMO pump is kept saline-primed in the ICU at all times, allowing initiation of extracorporeal support within 15 minutes. Precannulation support times dropped from an average of 90 minutes but still remained high at an average of 50 minutes. Of the remaining 11 patients, 10 were decannulated successfully, with 6 long-term survivors, 5 of whom were in New York Heart Association class I. This rapid deployment strategy likely will become more commonplace in large pediatric centers.
Under current 2010 AHA guidelines, centers should consider extracorporeal membrane oxygenation CPR for in-hospital cardiac arrest refractory to standard resuscitation attempts if the condition leading to cardiac arrest is reversible or amenable to heart transplantation, if excellent conventional CPR has been performed after no more than several minutes of no-flow cardiac arrest, and if the institution is able to rapidly perform ECMO. Long-term survival has been reported even after more than 150 minutes of CPR in selected patients.112
Data are emerging involving the role of ECMO in persons with refractory ventricular fibrillation.113 These data have solely been in the form of case reports but may represent a future direction for the care of patients with VF. In 2006, Samson et al.114 reported successful treatment of in-hospital VF in children with ECMO after cardiac arrest who had an initial rhythm of VF and immediate initiation of CPR.
Transcutaneous Cardiac Pacing
Transcutaneous cardiac pacing (TCP) is used as a method for noninvasive pacing of the ventricles for a relatively short period. Emergency cardiac pacing is successful in resuscitation only if it is initiated soon after the onset of arrest. In the absence of in situ pacing wires or an indwelling transvenous or esophageal pacing catheter, TCP is the preferred method for temporary electrical cardiac pacing. Since 1992, the AHA advanced cardiovascular life support (ACLS) guidelines have recommended the early use of an external pacemaker in patients with symptomatic bradycardia or asystole.115
Since Zoll116 established TCP in 1952 as a clinically useful method of pacing adult patients during ventricular standstill (Stokes-Adams attacks) and bradycardia-associated hypotension, numerous anecdotal reports have supported its use for bradycardic or asystolic arrests. Zoll et al.117 reported successful in-hospital resuscitation of 12 of 16 patients with hypotensive bradycardia or asystole if TCP was initiated within 5 minutes of the arrest. In contrast, if TCP was started between 5 and 30 minutes after the arrest, only 8 of 44 patients with either of these rhythms could be resuscitated.117 In two controlled clinical trials of prehospital TCP, no differences in the survival rate or success of resuscitation were observed in paced and nonpaced patients who had asystole or PEA.118,119 In patients with symptomatic bradycardia, TCP improved resuscitation and the survival rate.120
To date the efficacy of TCP in resuscitation of children has not been studied. Beland et al.121 showed that effective TCP could be achieved in hemodynamically stable children during induction of anesthesia for heart surgery. They were successful in 53 of 56 pacing trials, and the patients experienced no complications.121
To set up pacing, one electrode is placed anteriorly at the left sternal border and the other posteriorly just below the left scapula. Smaller electrodes are available for infants and children; adult-sized electrodes can be used in children weighing more than 15 kg.121 Electrocardiographic leads should be connected to the pacemaker, the demand or asynchronous mode selected, and an age-appropriate heart rate used. The stimulus output should be set at zero when the pacemaker is turned on and then increased gradually until electrical capture is seen on the monitor. The output required for a hemodynamically unstable rhythm is higher than that for a stable rhythm in children in whom the mean stimulus required for capture was between 52 and 65 mA. After electrical capture is achieved, one must ascertain whether an effective arterial pulse is generated. If pulses are not adequate, other resuscitative efforts should be used.
The most serious complication of TCP is induction of a ventricular arrhythmia.122 Fortunately, this complication is rare and may be prevented by pacing only in the demand mode. Mild transient erythema beneath the electrodes is common. Skeletal muscle contraction can be minimized by using large electrodes, a 40-ms pulse duration, and the smallest stimulus required for capture. Sedatives or analgesics may be necessary in the patient who is awake. If defibrillation or cardioversion is necessary, one must allow a distance of 2 to 3 cm between the electrode and paddles to prevent arcing of the current.
Pharmacology
Adrenergic Agonists
In 1963, only 3 years after the original description of closed-chest CPR, Pearson and Redding123 described the use of adrenergic agonists for resuscitation. They subsequently showed that early administration of epinephrine in a canine model of cardiac arrest improved the success rate of CPR. They also demonstrated that the increase in aortic diastolic pressure by administration of α-adrenergic agonists was responsible for the improved success of resuscitation. They theorized that vasopressors such as epinephrine were of value because the drug increased peripheral vascular tone, not because of a direct effect on the heart.124
Yakaitis et al.125 investigated the relative importance of α- and β-adrenergic agonist actions during resuscitation. Only 27% dogs that received a pure β-adrenergic receptor agonist along with an α-adrenergic antagonist were resuscitated successfully, compared with all of the dogs that received a pure α-adrenergic agonist and a β-adrenergic antagonist (Figure 33-12). Later studies reconfirmed this finding. Michael et al.37 demonstrated that the α-adrenergic effects of epinephrine result in intense vasoconstriction of the resistance vessels of all organs of the body, except those supplying the heart and brain. Because of the widespread vasoconstriction in nonvital organs, adequate perfusion pressure and thus blood flow to the heart and brain can be achieved despite the fact that cardiac output is very low during CPR (Figure 33-13).50
The increase in aortic diastolic pressure associated with epinephrine administration during CPR is critical for maintaining coronary blood flow and enhancing the success of resuscitation. Even though the contractile state of the myocardium is increased by use of β-adrenergic agonists in the spontaneously beating heart, during CPR, β-adrenergic agonists actually may decrease myocardial blood flow by increasing intramyocardial wall pressure and vascular resistance. This decrease in mycoardial blood flow could redistribute intramyocardial blood flow away from the subendocardium, increasing the likelihood of ischemic injury to this region.126 Moreover, evidence indicates that left ventricular end-diastolic pressure (LVEDP) rises with epinephrine use, reducing the overall impact of the vasoconstrictor effects of epinephrine on CPP. Tang et al.127 showed elevated LVEDP and decreased measures of diastolic performance in epinephrine-resuscitated rats after induced VF compared with phenylephrine-resuscitated animals or epinephrine-resuscitated animals who also received a β-blocker. Similar data were found by McNamara, who used a rat pup model of asphyxial arrest. LVEDP was increased and diastolic function indices decreased with epinephrine compared with either saline solution alone or epinephrine combined with verapamil. These data imply that excessive β-adrenergic effects prevent the intracellular calcium reuptake during diastole that is required for myocardial relaxation. By its inotropic and chronotropic effects, β-adrenergic stimulation increases myocardial oxygen demand, which, when superimposed on low coronary blood flow, increases the risk of ischemic injury. This combination of increased oxygen demand by β-adrenergic agonists128 and decreased oxygen supply may damage an already ischemic heart, raising the question of whether a pure α-adrenergic agonist would be better than epinephrine, which has significant β-adrenergic effects (Box 33-1). The effects on energy utilization and oxygen supply not only have implications for the success of the initial resuscitation but also for the postresuscitation function of the myocardium.
A number of studies have attempted to settle this controversy and actually have shown that pure α-adrenergic agonists can be used in place of epinephrine during CPR. Phenylephrine50,124,125 and methoxamine are two pure α-adrenergic agonists that have been used in animal models of CPR with success equal to that of epinephrine. More recently, vasopressin has been studied as a noncatecholamine vasoconstrictor in the management of patients who experience cardiac arrest.12 This agent is discussed in the section on vasopressin. These agents cause peripheral vasoconstriction and increase aortic diastolic pressure, resulting in improved myocardial and cerebral blood flow. This effect results in a higher oxygen supply/demand ratio in the ischemic heart and, at least, a theoretical advantage over the combined α- and β-adrenergic agonist effects of epinephrine. These agonists,124,130 as well as vasopressors such as vasopressin,12 have been used successfully for resuscitation. These drugs maintain blood flow to the heart during CPR as well as epinephrine does. In an animal model of VF cardiac arrest, a resuscitation rate of 75% was reported for both epinephrine- and phenylephrine-treated groups. In this study, the ratio of endocardial to epicardial blood flow was lower in the group treated with epinephrine, suggesting the presence of subendocardial ischemia.50 However, studies of this kind are difficult to interpret because of the inability to measure the degree of α-receptor activation by the different vasopressors. The higher subendocardial blood flow in the phenylephrine group may have been the result of less α-receptor activation.131–133 Moreover, some investigators have questioned the merits of using a pure α-adrenergic agonist during CPR. Although the inotropic and chronotropic effects of β-adrenergic agonists may have deleterious hemodynamic effects during CPR administered for VF, increases in both heart rate and contractility increase cardiac output when spontaneous coordinated ventricular contractions are achieved.
Cerebral blood flow during CPR, like coronary blood flow, depends on peripheral vasoconstriction and is enhanced by use of α-adrenergic agonists. This action produces selective vasoconstriction of noncerebral peripheral vessels to areas of the head and scalp without causing cerebral vasoconstriction.50 As with myocardial blood flow, pure α-agonist agents are as effective as epinephrine in generating and sustaining cerebral blood flow during CPR in adult animal models130 and in infant models (Figure 33-14).50 No difference in neurologic deficits 24 hours after cardiac arrest was found between animals receiving either epinephrine or phenylephrine during CPR.134
Analogous to the heart, β-adrenergic agonists could increase cerebral oxygen uptake if a sufficient amount of drug crosses the blood-brain barrier during or after resuscitation. In addition, adrenergic agonists may vasoconstrict or dilate cerebral vessels, depending on the balance between α- and β-adrenergic receptors. Epinephrine and phenylephrine had similar effects on cerebral blood flow and metabolism, maintaining normal cerebral oxygen uptake for 20 minutes of CPR in dogs. This finding implies that cerebral blood flow was high enough to maintain adequate cerebral metabolism and that β-receptor stimulation did not increase cerebral oxygen uptake, despite the fact that the combined effects of brain ischemia and CPR can increase the permeability of the blood-brain barrier to drugs used during CPR or when enzymatic barriers to vasopressors (e.g., by monoamine oxidase) are overwhelmed during tissue hypoxia. Mechanical disruption of the barrier could occur during chest compressions by large fluctuations in cerebral venous and arterial pressures or as a result of hyperemia, the large increase in cerebral blood flow that occurs during the early reperfusion period when the cerebral vascular bed is maximally dilated following resuscitation, particularly if systemic hypertension occurs.135 No blood-brain barrier permeability changes during CPR immediately after resuscitation or 4 hours after resuscitation were found in adult dogs.135 However, after 8 minutes of cardiac arrest and 6 minutes of CPR in piglets, the blood-brain barrier was permeable to the small neutral amino acid α-aminoisobutyric acid 4 hours after cardiac arrest (Figure 33-15).136 The increase in permeability could be prevented by prearrest administration of conjugated superoxide dismutase and catalase,137 indicating a role of oxygen free radicals in the pathogenesis of this injury to the blood-brain barrier (Figure 33-16). These endothelial membrane changes frequently were associated with the presence of intravascular polymorphonuclear and monocytic leukocytes.138 Whether leukocytes disrupt the blood-brain barrier by release of toxic substances, such as oxygen free radicals or proteases, or appear in the postischemic microvessels as an epiphenomenon of a more important derangement is unknown (Figure 33-17).
< div class='tao-gold-member'>
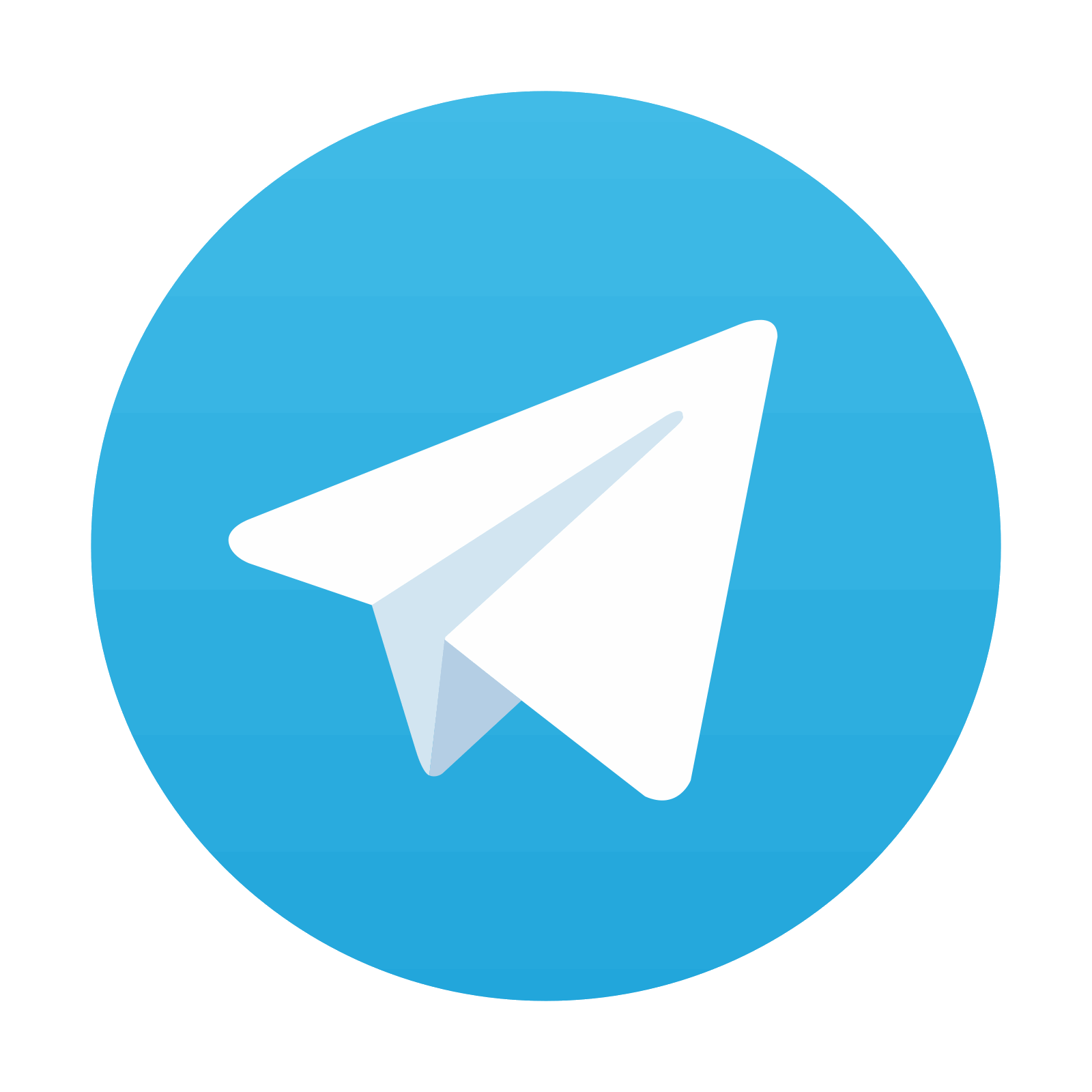
Stay updated, free articles. Join our Telegram channel
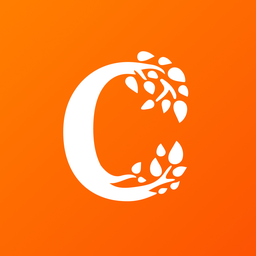
Full access? Get Clinical Tree
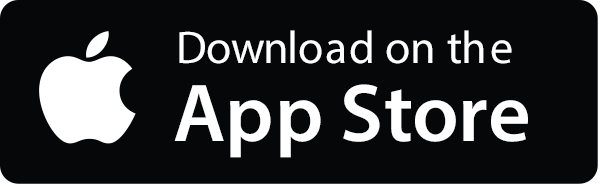
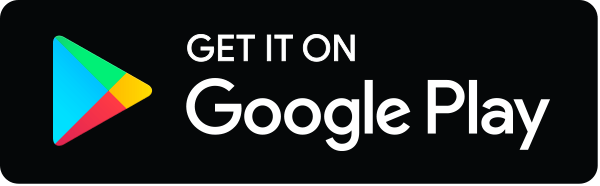