Chapter Outline
PEDIATRIC ANESTHESIA EQUIPMENT
SUPRAGLOTTIC AIRWAYS IN PEDIATRIC PATIENTS
Fundamental Requirements of the Pediatric Breathing System
Mapleson D, E, and F System Development
Factors that Affect Delivered Tidal Volume
Miscellaneous Aspects of Mechanical Ventilation
Practical Hints for Ventilating Neonates and Infants
Manual Versus Mechanical Ventilation of Neonates and Infants
Summary of Mechanical Ventilation of Infants and Children During Anesthesia
Beneficial Effects of Humidification
Reduction of Heat and Water Loss from the Respiratory Tract
Prevention of Respiratory Tract Mucociliary Dysfunction
Methods of Providing Humidification in Pediatric Systems
CAPNOGRAPHY AND RESPIRATORY GAS ANALYSIS IN PEDIATRIC PATIENTS
Overview
The ultimate goal of the anesthesiologist during pediatric surgery is to provide the patient with a safe and smooth anesthetic along with a comfortable and uneventful recovery. A well-considered anesthetic plan for a pediatric patient must include a well-informed choice of appropriate equipment. Which breathing systems are appropriate for the pediatric patient? How do maturational changes in respiratory physiology affect the selection and use of equipment? Does an adult ventilator and breathing system function acceptably for the pediatric patient? Are monitors primarily designed for adults appropriate for pediatric patients? This chapter attempts to answer some of these questions.
A clear understanding of anesthetic equipment is important to the safe delivery of pediatric anesthesia. Approximately 40% of anesthetic incidents were attributed to equipment problems in one 11-year experience, which included both adult and pediatric patients. Standardization of equipment, improved monitoring, and the introduction of new anesthetic drugs have all improved the safety of pediatric anesthesia. Over time, the percentage of pediatric closed claims events attributable to respiratory events or to inadequate monitoring have declined.
Physiology
Work of Breathing Under Anesthesia
Understanding the relevant aspects of pediatric respiratory physiology provides a rationale for the recommendations presented later in this chapter. Several factors may increase the work of breathing under anesthesia ( Table 17-1 ). These factors can be broadly divided into two groups: first is the increased work of breathing that may be imposed by the anesthesia breathing system, including inspiratory and expiratory resistance of breathing circuits, circuit dead space, and rebreathing of carbon dioxide. Second are changes in the mechanics and in the control of breathing induced by general anesthesia. These include decreased total respiratory compliance, decreased lung volume, airway closure, and upper airway obstruction. Thus, during general anesthesia, many factors conspire to increase the demands placed on the respiratory system while simultaneously decreasing the patient’s ability to cope with these demands.
Anesthetic Breathing System | General Anesthesia |
---|---|
Circuit and tube resistance | Decreased lung volume |
Absorber resistance | Decreased respiratory compliance |
Valvular resistance | Upper airway obstruction |
Apparatus dead space Rebreathing of carbon dioxide |
Table 17-2 compares some selected infant and adult respiratory physiologic values. In general, neonates and infants have less respiratory reserve than adults and may demonstrate an unpredictable response to any additional work of breathing through a circuit under general anesthesia. Patients breathing spontaneously with the Mapleson D, E, and F circuits will rebreathe alveolar gas if the fresh gas flow is less than 2.5 to 3 times the predicted minute ventilation. Under normal circumstances, most of these patients will increase their minute ventilation in response and thereby prevent significant hypercarbia. However, even some anesthetized adults are unable to respond to increases in inspired carbon dioxide by hyperventilation and thus become significantly hypercarbic.
Normal Value | Infant | Adult |
---|---|---|
Weight (kg) | 3.0 | 70 |
Surface area (m 2 ) | 0.2 | 1.8 |
Surface area/weight (m 2 /kg) | 0.06 | 0.03 |
Respiratory frequency (breaths/min) | 30-40 | 10-16 |
Tidal volume (V T , mL/kg) | 7 | 7 |
Dead space (V D , mL/kg) | 2.2 | 2.2 |
V D /V T | 0.3 | 0.3 |
Functional residual capacity (mL/kg) | 30 | 30 |
Specific compliance (mL/cm H 2 O/mL) | 0.05 | 0.05 |
Airways resistance (cm H 2 O/L/sec) | 25-30 | 1.6 |
Work of breathing (J/L) | 0.5-0.7 | 0.5-0.7 |
Alveolar ventilation (mL/kg/min) | 100-150 | 70 |
Oxygen consumption (mL/kg/min) | 6 to 8 | 4 |
Olsson and Lindhal examined the response of spontaneously breathing anesthetized infants (nitrous oxide and 1% to 1.5% halothane) to an increase in inspired carbon dioxide. Patients younger than 6 months did not significantly increase their minute ventilation in response to the added carbon dioxide, whereas patients older than 6 months showed a markedly attenuated response to increased inspired carbon dioxide. When the halothane concentration was reduced to 0.8%, the ventilatory response to carbon dioxide returned in patients younger than 6 months; thus, inhalational anesthesia attenuates the ventilatory response to carbon dioxide in small infants.
General anesthesia also adversely affects infants’ ventilatory response to increased respiratory work in the forms of tubular resistance, dead space, and valvular resistance. For a given increase in apparatus dead space, the infant must increase minute ventilation to a proportionately greater degree to maintain normocarbia when compared with the older child. The neonate is prone to respiratory compromise in the face of prolonged increases in respiratory work. The newborn’s diaphragmatic muscle is immature, having a smaller proportion of fatigue-resistant high-oxidative muscle fibers. In addition, the infant diaphragm is less able to generate a maximal inspiratory force compared with the adult diaphragm.
Pediatric Anesthesia Equipment
Face Masks
The face mask must be able to provide a good seal to the child’s face to facilitate effective positive-pressure ventilation, avoid entrainment of room air, and reduce operating room (OR) pollution. Other desirable face mask features include low dead space, increased patient comfort, and construction with transparent material that allows observation of patient color and the presence of secretions or vomitus ( Fig. 17-1 ); in addition, transparent masks are less threatening to the patient. The ability to ventilate the patient by mask is of the utmost importance, but low dead space is also desirable to avoid retention of carbon dioxide and increased respiratory work. However, in practice, the functional dead space of most face masks is significantly less than the measured dead space because streaming of gas eliminates significant rebreathing.

Maintaining an airway with a face mask occasionally can be challenging in children because the anesthesiologist’s fingers can cause external compression of the compliant upper airway. Care should be taken to ensure that fingers do not stray from the mandible onto the soft tissues surrounding the airway. Mask anesthesia with spontaneous ventilation should probably not be used for prolonged procedures in small infants because infants are unable to tolerate the increased work of breathing and may respond with apnea or hypoventilation. Inhalational induction of anesthesia may be facilitated by the application of an odorant (e.g., lip gloss) to the inside of the face mask to disguise the smell of the potent volatile agents and the unpleasant plastic odor of the mask and breathing circuit.
Tracheal Tubes
Selection of the correct tracheal tube type and size, along with its accurate and secure positioning, is vitally important.
Cuffed Versus Uncuffed Endotracheal Tubes
The decision to use cuffed instead of uncuffed endotracheal tubes (ETTs) in pediatric patients depends on multiple factors. The use of cuffed tubes has historically been discouraged in children younger than 8 to 10 years. Most pediatric anesthesiologists have strong opinions on this matter, and evolving practice patterns are not always evidence driven. Although this topic generates vigorous debate, irrespective of whether a cuffed or uncuffed tube is selected, most experts would agree that safe use of any tube depends on meticulous attention to technical detail.
The vocal cords are the narrowest point of the pediatric airway, but they are more mobile that the cricoid cartilage, which completely surrounds the airway. Although the cricoid often is described as a “ring” of cartilage, the airway at this level has an ellipsoid cross-section. Intuitively, it would seem that the ellipsoid cross-sectional configuration of the airway makes it unlikely that a circular, cross-sectional tube can both provide an optimal airway seal and avoid local trauma to some part of the airway circumference, particularly the posterolateral walls of the larynx. However, the subglottic region is lined with loosely bound pseudostratified epithelium. Thus, trauma to this region, such as by intubation with a tube of too large a diameter, readily results in edema that decreases the lumen of the airway and increases resistance to gas flow. During laminar flow, resistance is inversely proportional to the fourth power of the radius. However, flow conditions in the upper airway are likely to be transitional or turbulent. Under these conditions, resistance is inversely proportional to the fifth power of the radius. A 1-mm ring of edema in the newborn will reduce the cross-sectional area of the airway by 75%. Under laminar flow conditions, resistance will increase 16-fold. By comparison, a 1-mm ring of edema in a healthy adult would have a less significant effect on airway resistance.
Avoiding trauma to the vulnerable subglottic region is obviously critical. This applies whether the insult is generated by the shaft of an uncuffed tube or from an ETT cuff. Therefore, an important consideration of pediatric ETTs is their cuff design characteristics. Over time, cuff pressure-volume characteristics have led to the development of less traumatic high-volume low-pressure cuffs. However, recent attempts have been made to eliminate cuff contact with the subglottic region altogether by locating a shorter, ultrathin polyurethane cuff more distally on the shaft of the tube, thus producing a cuff-free subglottic zone. The more distal cuff location has forced the elimination of the Murphy eye. The intent of this design modification is to create a tracheal, rather than a cricoid, cuff seal. The cross-sectional configuration, presence of a nonrigid muscular posterior wall, and U-shaped cartilage make the trachea a more suitable site for cuff placement than the cricoid region. Modern high-volume, low-pressure, ultrathin polyurethane cuffs conform readily to the trachea and provide acceptable seals at lower pressures (<15 cm H 2 O).
We prefer to use cuffed ETTs in our practice. However, the use of cuffed tubes is predicated on the availability of tubes of the appropriate design and careful attention to leak pressure. Use of cuffed tubes must be accompanied by careful attention to cuff pressure and may require using a half-size smaller tube to provide space for the uninflated cuff. However, these limitations are offset by several advantages: first, use of a cuffed tube with appropriate inflation provides a reliable airway seal, reduces the risk of aspiration, and limits inspired gas leakage. Second, the improved seal also improves tidal volume measurement and the accuracy of airway gas samples. Third, contamination of the OR ambient atmosphere by anesthetic gas is reduced. Fourth, more consistent ventilation and better carbon dioxide management particularly benefits patients with congenital heart disease or pulmonary hypertension.
Uncuffed Tubes
The optimal size of an uncuffed tracheal tube is the largest size that will easily pass into the trachea without traumatizing the larynx. To determine the leak pressure after tracheal intubation, the reservoir bag should be slowly inflated; circuit pressure is measured while listening with a stethoscope, either at the mouth or in the sternal notch, for a leak. The optimal leak pressure has not been definitively determined, but the incidence of postextubation stridor seems to increase as the leak pressure increases, particularly to greater than 25 cm H 2 O. Most patients’ lungs can be adequately ventilated at leak pressures of 15 to 20 cm H 2 O. If the patient has lung disease or is undergoing an intrathoracic or high abdominal procedure, a higher leak pressure, such as 25 to 30 cm H 2 O, may be necessary to allow adequate ventilation in the face of decreased respiratory compliance. On occasion, an unacceptably large leak will be present with one size of tube but not with a tube 0.5 mm larger in diameter. Under these circumstances, a cuffed tube of the smaller size with the cuff deflated may produce an acceptable leak. Using a tube that is too small with a large leak may lead to difficulty ventilating the lungs and obtaining accurate end-tidal carbon dioxide measurements and, at least in theory, it increases the risk of aspiration. Suction catheters appropriate to all tube sizes must be immediately available to remove secretions from tracheal tubes.
Formulas to predict correct tracheal tube size are based on weight and age ( Table 17-3 ). It is also good practice to also have tubes available sized 0.5 mm above and below the predicted size ( Fig. 17-2 ).
Patient Age | Tube Size | Depth |
---|---|---|
Premature, ≤1000 g | 2.5 mm ID | 7 cm for a 1-kg infant: +1 cm/kg body weight up to 4 kg |
Premature, 1000-2500 g | 3.0 mm ID | >4 kg and ≤1 year: 10 cm at the alveolar ridge |
<6 years | (Age/3) + 3.75 mm ID | >1 year: (age/2) + 12 cm |
>6 years | (Age/4) + 4.5 mm ID |

The peak incidence of postextubation stridor is between 1 and 4 years of age. Factors that contribute to the development of postextubation stridor include a tight-fitting tracheal tube (i.e., a leak at >25 cm H 2 O), traumatic or multiple intubations, coughing with the tracheal tube in place during emergence from anesthesia, changing the patient’s head position while intubated, intubation lasting more than 1 hour, and operations in the neck region. Although recent upper respiratory tract infection was not initially thought to be a contributing factor, more recent data show that patients younger than 1 year have a 27-fold increased risk of postextubation stridor after upper respiratory tract infection. Patients with Down syndrome may have a narrow subglottic region and are thus prone to postextubation stridor; the clinician should consider using an ETT smaller than that predicted by the patient’s age or weight for such patients.
Selecting the Correct Insertion Depth
The term newborn trachea is approximately 4 to 5 cm long. Therefore, the margin of error for a tube located in the mid trachea is approximately 2 cm in either direction. A tube inserted too deeply will result in carinal irritation or endobronchial intubation, whereas too shallow an insertion depth may predispose to accidental extubation. A potential hazard of shallow tube insertion depth is that the cuff may be located in the subglottic, rather than the tracheal, region. To determine the correct insertion depth, gently advance the tube after intubation, while the chest is auscultated bilaterally, to determine the depth at which bronchial intubation occurs. The tube is then carefully withdrawn and secured at the appropriate depth. Of note, when the tube has a Murphy eye, it may be difficult to determine tube position by auscultation because sufficient gas may flow through the eye to produce contralateral breath sounds despite the tube tip being located in a mainstem bronchus.
In small infants, particularly those with lung disease, it is not always easy to determine by auscultation alone at what depth the tube should be secured. When accurate tube position is crucial (e.g., for repair of a tracheoesophageal fistula), a small-diameter flexible fiberoptic bronchoscope may be used to determine the tube location, and 1.8 mm (Olympus PF 18M; Olympus America, Center Valley, PA) and 2.2 mm (Olympus LF-P) outer diameter (OD) flexible fiberoptic bronchoscopes can help in tube positioning. The larger of these bronchoscopes has a directable distal tip. Neither has a suction channel, but both can be passed through a 2.5-mm internal diameter (ID) tracheal tube. If a suitable bronchoscope is unavailable, a chest radiograph may be a reasonable alternative.
Several manufacturers place intubation depth markers on their ETTs. These markers typically are one or more horizontal black bands located near the distal end of the tube. The intent is that when the marker is placed at the vocal cords, the distal tip of the ETT will be located in the mid trachea. Goel and colleagues examined intubation depth markers on several commonly used pediatric ETTs and noted a wide discrepancy in both the number and locations of the markers among manufacturers. Furthermore, a lack of consistency of markers was found among different-sized tubes from the same manufacturer. Thus it is important to be familiar with the location of the intubation depth markers on the tubes most frequently used at an institution. Optimal insertion depth is probably best achieved by using a combination of predictive formulas (see Table 17-3 ), intubation depth markers, and clinical examination.
Using the Oral RAE Tracheal Tube
The preformed Ring-Adair-Elwyn tube (RAE; Mallinckrodt, St. Louis, MO) (see Fig. 17-2 ) is often used during ear-nose-throat, plastic, and other head and neck procedures. The RAE tube has several advantages and potential pitfalls: it was originally designed for use during cleft lip and palate surgery at an institution where the surgeon preferred to leave the tube untaped so that its position could be adjusted during the procedure. The designers of the tube intended the distal tip of the tube to lie nearer to the carina than a conventional tube to reduce the likelihood of accidental extubation. Two Murphy eyes, located at the distal end of the tube, are intended to allow continued ventilation should endobronchial migration occur. The acute angle in the RAE tube, the part of the tube most likely to kink, is located outside the mouth and therefore is readily visible.
Although the above design features are useful, several potential hazards have been described. Seemingly appropriate positioning of the acute angle of an RAE tube at the lower lip does not guarantee that the distal tip of the tube is located correctly. The distal tips of 32% of RAE tubes positioned in this way were located at the carina or down a mainstem bronchus. In this situation, the Murphy eyes do not guarantee adequate ventilation during the procedure, but they may allow sufficient gas flow during manual ventilation and chest auscultation to falsely suggest acceptable tube position. It is recommended that a left precordial stethoscope be used to monitor tube position throughout the procedure, particularly during repositioning and mouth-gag insertion, to rapidly detect endobronchial migration. These tubes are labeled by the manufacturer with a suggested patient age range, but these recommendations should be interpreted with caution, as they may advocate a different-sized tube than that predicted by standard formulas for pediatric tracheal tubes.
It should be noted that, although unusual in current practice, occasional patients who require cleft lip and/or palate repair may be smaller than predicted by age, typically as a result of feeding difficulties or coexisting disease. In these patients it can be challenging to select an ETT size that is appropriate in both diameter and length. However, in the majority of patients with cleft lip and palate, standard formulas are acceptable predictors of ETT size and insertion depth.
Another obstacle to correct tube sizing is related to significant differences among manufacturers in the dimensions of oral preformed tubes. For example, when 4.5-mm ID uncuffed tubes from four manufacturers were measured, the distance from the bend to the tube tip ranged between 13.5 and 15 cm.
Problems may occasionally be encountered when performing tracheal suctioning via an RAE tube, and passing a catheter beyond the acute angle in the tube can be difficult. Despite these potential problems, and with attention to the details noted above, anesthesiologists have found the RAE tube to be extremely useful during procedures of the head and neck.
Supraglottic Airways In Pediatric Patients
The laryngeal mask airway (LMA; LMA North America, San Diego, CA) revolutionized airway management for the practice of anesthesia ( Fig. 17-3 ). The LMA provides a more secure airway than a face mask and pharyngeal airway but is less invasive than a tracheal tube. The success of the original LMA design has been underscored by the large number of supraglottic airway variants that have been developed and marketed by other manfacturers. The creation of a separate category of airway devices, the supraglottic airways, is also indicative of the importance of these devices to airway management. Although originally developed for the management of normal airways, the LMA has proven extremely useful for management of difficult airways.

Pediatric patients are especially suited for supraglottic airways, because upper airway obstruction during anesthesia is more likely in children than in adults. Children often require general anesthesia for short surgical or diagnostic procedures, and their small size can place the anesthesiologist at a considerable distance from the patient’s head and airway. Prior to the introduction of the LMA, tracheal intubation was often required for such procedures. Compared with tracheal intubation, advantages of the supraglottic airway include less hemodynamic response to insertion and, during emergence, a reduced risk of laryngeal complications such as croup; a decreased incidence of sore throat; and, quite possibly, less nausea and a faster discharge from the postanesthesia care unit (PACU).
Disadvantages of supraglottic airways in children are generally related to malposition of the device, excessive pressure on the oropharyngeal mucosa, hypoventilation, laryngospasm, and aspiration of gastric contents. A light plane of anesthesia may predispose to regurgitation or laryngospasm with a supraglottic airway in place.
Education and the Supraglottic Airway
Airway management during the administration of anesthesia requires precise positioning of the supraglottic airway to maximize ventilatory exchange and minimize the risk of hypoventilation, laryngospasm, and mechanical distortion of the upper airway. Instruction of supraglottic airway use to anesthesiology trainees must be methodical and controlled and must permit adequate experience for the trainee to understand the indications and contraindications for use. Fiberoptic examination of the supraglottic airway after insertion is invaluable for anyone learning to see position variation and how malposition may adversely affect airway function. Experience with different types of supraglottic airways is important for trainees because different institutions use different types.
Insertion Technique
The original insertion technique for LMA insertion has been modified by many users during the past 30 years. Ideal insertion of a supraglottic airway places the airway channel immediately above the glottic inlet, with the distal end resting in the upper esophageal sphincter and the upper border of the device bowl below the hyoid bone. The airway should slide along the hard and soft palates, between the tonsillar pillars, and into the hypopharynx. Insertion techniques for supraglottic airways with cuffs have been described with the cuff completely deflated, partially inflated, and completely inflated ( Fig. 17-4 ). The potential problem with insertion with the cuff inflated is that the epiglottis will fold downward, and the device will be high in the hypopharynx, thereby increasing pressure on the base of the tongue and the lateral oropharyngeal wall. Insertion of a supraglottic airway upside-down and partially inflated, with rotation of the device after it enters the pharynx, has been advocated by many authors. Large inflation volumes may cause a greater incidence of sore throat and increase the risk of excessive pressure on the pharyngeal mucosa.

An adequate depth of anesthesia must be attained prior to supraglottic airway insertion. Insertion of the device before attenuation of upper airway reflexes may provoke emesis or regurgitation. Satisfactory anesthesia can be achieved with both intravenous (IV) and inhaled agents.
Types of Supraglottic Airways
Several manufacturers produce supraglottic airways, and product lines continue to evolve ( Box 17-1 ). Many supraglottic airways do not undergo rigorous evaluation prior to clinical introduction, and potential complications do not become known until enough patient uses have been achieved. Consequently, some supraglottic airways have a very short market life. Most institutions now use single-use (disposable) supraglottic airways to minimize the risk of infectious disease transmission and to reduce processing costs.
Laryngeal Mask Airway (LMA North America, San Diego, CA)
air-Q (Mercury Medical, Clearwater, FL)
Ambu Airway (Ambu Inc., Ballerup, Denmark)
I-Gel (Intersurgical, Liverpool, NY)
Some supraglottic airways may be especially suited for a particular procedure, and it is unlikely that one supraglottic airway will fit all needs. Flexible bronchoscopy is easily performed through a supraglottic airway, but bronchoscope manipulation is easier through a silicone airway (e.g., classic reusable LMA). The pediatric anesthesiologist should be facile with several types of supraglottic airways to confront a wide array of patient airways.
Laryngeal Mask Airway
Since its introduction into clinical practice in the late 1980s, several models of the LMA have been developed ( Box 17-2 ). In addition to the LMA Classic, single-use LMAs (LMA Unique), flexible LMAs, intubating LMAs (Fastrach), the ProSeal LMA, and Supreme LMA are currently available. The Fastrach LMA is noted for its ease of insertion and for the fact that it can serve as both a conduit for ventilation and tracheal intubation. The intubating LMA is available only in sizes 3, 4, and 5. All other models are available in smaller sizes. The LMA C Trach is an advanced model of the LMA Fastrach intubating LMA that has imaging capability of the larynx after insertion of the LMA. Although the C Trach has been most frequently used in adults, successful pediatric experience has been reported for children aged 9 to 17 years. The ProSeal LMA and the LMA Supreme are perhaps the most advanced of the LMAs and consist of a more anatomically designed bowl that results in a higher leak pressure without excessive pressure on the hypopharynx. A gastric conduit is built into the LMA Supreme and the ProSeal LMA and permits gastric decompression.
LMA Classic
LMA Unique
LMA Flexible
LMA Fastrach
LMA ProSeal
LMA Supreme
The medical literature regarding the LMA is extensive and documents its utility in pediatric patients with normal and difficult airways.
air-Q
The air-Q intubating laryngeal airway (Mercury Medical, Clearwater, FL) is a supraglottic airway that can be used by itself or as a conduit for tracheal intubation. It is available in sizes to fit a wide range of patients from neonates to large adults. One recent version, the ILA-SP, is self-pressurized. A study of 352 patients, aged newborn to 18 years, reported a mean leak pressure of 20.4 cm H 2 O, complications in 14 patients, and no cases of regurgitation or aspiration. Successful placement on the first attempt was performed in 95% of the patients. Tracheal intubation via the air-Q can be performed efficiently and effectively with guidance from a flexible fiberscope.
Ambu Airway
There are five models of the Ambu laryngeal airway: the AuraFlex, AuraStraight, AuraOnce, Aura-I, and Aura40 (Ambu Inc., Ballerup, Denmark). The AuraFlex is available in sizes 2 through 6 with a small half size (2.5). All other models are available in sizes 1 through 6 with two small half sizes (1.5 and 2.5). The Aura40 is a curved reusable supraglottic airway, and the AuraOnce is a curved single-use supraglottic airway. Performance of the Ambu laryngeal airway compares favorably with other supraglottic airways, although few published studies have been done in pediatric patients.
I-Gel
The I-Gel (Intersurgical, Liverpool, NY) is a supraglottic airway with a laryngeal cuff composed of a thermoplastic elastomer that produces a perilaryngal seal without an inflatable cuff. The purpose of this design is to provide an adequate laryngeal seal while minimizing the risk of pressure trauma to the hypoharyngeal tissue. A bite block and gastric drain port are also built into the device to permit decompression of the stomach. Reported experience with the I-Gel in children is limited but indicates a high insertion success rate and a mean leak pressure of 25 cm H 2 O. There are 7 sizes of the I-Gel that cover patients weighing 2 kg to more than 90 kg.
Pediatric Breathing Systems
The preterm infant can be adversely affected by hyperoxia. Consequently, no matter what breathing system is used, it is important to control the delivered oxygen concentration, which may be room air. The modern anesthesia machine can be used very successfully for most pediatric cases and has the great advantage of operator familiarity. However, it is very important that the machine have the capability of delivering air.
Fundamental Requirements of the Pediatric Breathing System
The pediatric breathing system should have a low resistance and low dead space, be efficient for use with both spontaneous and controlled ventilation, and be easy to humidify and scavenge ( Box 17-3 ). If the circuit contains valves, they should offer minimal resistance to gas flow and must be reliable. In addition, the circuit should have a low compressible volume and be lightweight and compact. The circle system with carbon dioxide absorbent is commonly used for pediatric anesthesia, as is the Mapleson D and F systems for manual ventilation and in PACU settings.
Low dead space
Low resistance
Lightweight and compact
Low compressible volume
Easily humidified
Easily scavenged
Suitability for both controlled and spontaneous ventilation
Economy of fresh gas flow
Mapleson D, E, and F System Development
The Mapleson D, E, and F systems are direct descendants of the original T-piece system introduced in 1937 by Philip Ayre of Newcastle, England. The original Ayre T-piece has been greatly modified over the years and is rarely used today. All have the common feature of containing no valves that direct gas flow toward or away from the patient. The most commonly used configurations are the Mapleson D and F systems ( Figs. 17-5 and 17-6 ). The Mapleson F system is a modification that is generally attributed to Jackson Rees, who added a reservoir bag with an adjustable valve to the expiratory limb. When used with the valve largely open, the movement of the reservoir bag allows monitoring of spontaneous ventilation. With the valve partially closed, manual compression of the bag allows controlled ventilation. The Mapleson D system has an expiratory valve located at the end of the expiratory limb and functions in a manner similar to the Mapleson F circuit. The volume of the expiratory limb should exceed the patient’s tidal volume. The Mapleson D and F circuits can be used with mechanical ventilation by removing the reservoir bag and connecting a ventilator.


The absence of valves with the Mapleson systems can result in rebreathing of exhaled gases in a setting of decreased fresh gas flow (FGF). The use of capnometry to determine if there is rebreathing (PiCO 2 >0) allows titration of FGF to eliminate rebreathing if desired. In general, an FGF of not less than 2.5 and up to 3 times the minute ventilation will usually eliminate rebreathing ( Box 17-4 ). Neonates generally require an FGF of 3 L/min. FGF may need to be increased in the presence of a large tracheal tube leak. Significant FGF will be required in larger children and adults to prevent rebreathing, where peak inspiratory flows may exceed 20 L/min. FGF of this magnitude is both difficult to humidify and expensive. In general, a circle system is more efficient in terms of FGF requirements during anesthesia, particularly in older children and adults. A valved self-inflating breathing bag is appropriate for manual ventilation of larger children and adults when inhaled anesthetic delivery is not being used, particularly during patient transport.
Spontaneous Ventilation
Mask Anesthesia
<30 kg body weight:
FGF = 4 × (1000 + [100 × kg body weight])
>30 kg body weight:
FGF = 4 × (2000 + [50 × body weight])
Intubated
<30 kg body weight:
FGF = 3 × (1000 + [100 × kg body weight])
>30 kg body weight:
FGF = 3 × (2000 + [50 × body weight])
Controlled Ventilation
10-30 kg body weight:
FGF = 1000 mL + 100 mL/kg
>30 kg body weight:
FGF = 2000 mL + 50 mL/kg
The Bain circuit, described by Bain and Spoerel in 1972, is functionally identical to the Mapleson D system from which it is derived. In the Bain circuit, the FGF is carried in a smaller inner hose, contained within the expiratory limb, attached to the machine using a special adapter ( Fig. 17-7 ). The outer expiratory limb ID is 22 mm and is 7 mm for the inner fresh gas tubing. FGF requirements for the Bain system are similar to those recommended for the Mapleson D and F systems.

Before using the Bain circuit, it is important to verify that the fresh gas inner tube is not fractured or disconnected. In this situation, fresh gas will enter the expiratory limb near the reservoir bag, and a huge dead space will result. Pethick’s maneuver has been recommended to test the integrity of the circuit. First, the patient end of the circuit is occluded and the reservoir bag is filled. The patient end is then opened, and a high flow of oxygen is passed through the circuit using the oxygen flush valve. The high gas flow exiting from the patient end of an intact fresh gas hose will cause a reduction in pressure in the expiratory limb because of the Venturi effect, collapsing the reservoir bag. If the inner tube is disrupted, oxygen under pressure will enter the expiratory limb and distend the reservoir bag. This technique has been criticized because small leaks may go undetected, and the test itself may cause circuit disruption. Similarly, if the inner tube is intact but does not extend completely to the end of the expiratory limb, significant dead space will exist that may not be detected. An adapter has been described that tests the integrity of the inner and outer tubing separately ( Fig. 17-8 ). A similar malfunction of a breathing system caused by disconnection of the central coaxial tubing can occur with a coaxial circuit used with a circle system.
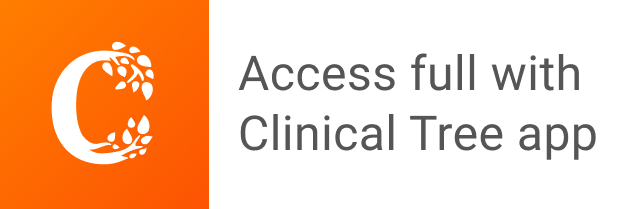