Chapter Outline
CLASSIFICATIONS OF BREATHING CIRCUITS
COMPONENTS OF A BREATHING CIRCUIT
Placement of the Carbon Dioxide Absorber, Fresh Gas Inflow, and Pop-off Valve
Semiclosed Systems: Mapleson Classification
Proprietary Semiclosed Systems
The Anesthesia Machine
The anesthesia machine serves to create a desired mixture of anesthetic gases, vapors, oxygen, and air (as well as other gases such as helium and carbon dioxide, albeit less frequently). The patient is the recipient of these prepared gas mixtures of known composition, and the breathing circuit is the interface between the anesthesia machine and the patient. This circuit delivers the gas mixture from the machine to the patient as it removes carbon dioxide, excludes operating room (OR) air, and conditions the gas mixture by adjusting its temperature and humidity. It converts continuous gas flow from the anesthesia machine to the intermittent flow of breathing, facilitates controlled or assisted respiration, and provides other functions such as gas sampling and pressure and spirometric measurements.
The desirable characteristics of a breathing circuit include 1) low resistance to gas flow, 2) minimal rebreathing of the preceding alveolar expirate, 3) removal of carbon dioxide at the rate at which it is produced, 4) rapid changes in delivered gas composition when required, 5) warmed humidification of the inspirate, and 6) safe disposal of waste gases. The components of a breathing circuit include the breathing tubing; respiratory valves; reservoir bags; carbon dioxide absorption canisters; a fresh gas inflow site; a pop-off valve leading to a scavenger for excess gas; a Y-piece with a mask or tube mount; and a face mask, laryngeal mask, or tracheal tube. Other devices that may be included are filters; humidifiers; valves for positive end-expiratory pressure (PEEP); and detecting mechanisms for airway pressure, spirometry, and gas analysis. Although these circuit components can be assembled in many ways, contemporary systems are usually configured by the manufacturer and permit little intervention by the user in regard to their configuration. Understanding the advantages and limitations of the different configurations allows the user to select the most appropriate type for varying clinical settings.
History of Device Development
Breathing circuits have been an important concern from the start. Because of a delay in the production of his inhaler, Morton was late to his first public exhibition of the “Somniferon” (ether) in 1846. The earliest circuits were mechanically simple; differences among them were related to the characteristics of the primary anesthetic agent. Because nitrous oxide and ether anesthetic mixtures were weak (less potent) or slow to produce anesthesia, it was necessary to exclude air and helpful to include oxygen enrichment. The rapid onset of action and potency of chloroform, on the other hand, demanded precise control. It became apparent that the unique features of each agent were important. The ability to assist respiration was advantageous, as was conservation of costly agents and avoidance of large leaks of flammable ones.
In the twentieth century, a large number of relatively small but more highly engineered improvements were made as other demands on the breathing circuit were recognized. In 1915, Dennis Jackson described the first carbon dioxide absorber to save on the cost of nitrous oxide for animal studies. Ralph Waters brought the idea into the OR, designing a to-and-fro absorption canister that used soda lime. Bryan Sword introduced the first circle breathing circuit in 1930. Thus low-flow absorption systems were already in use when cyclopropane made them essential. A return to high flows in the United States was brought about by the poor performance of vaporizers for halothane in the 1950s, with the demonstration that such flows could eliminate carbon dioxide without the use of soda lime.
Stimulated by Magill’s use of a number of pieces of apparatus put together in differing configurations for differing purposes, Mapleson described a variety of Magill circuits. The original Ayre’s T-piece was modified by numerous practitioners; the Jackson-Rees circuit represents one such example. A variety of proprietary nonrebreathing valves were introduced, and the circuits named for them included the Stephen-Slater, the Fink, the Ruben, and the Frumin.
Partial rebreathing and functionally nonrebreathing circuits—such as the Bain, Humphrey ADE, and Lack systems—found various proponents. Ingenious switching valves permitted transfiguration from one circuit to another, which led to difficulty in remembering which circuit was optimal for what purpose.
Today, in addition to factors of convenience and economy, circuits are used to control heat and humidity; to measure patient variables such as tidal volume, respiratory frequency, airway pressure, and inspired and expired gas concentrations; and to control contamination of the OR environment by the agents themselves. The 150-year history of the development of the breathing circuit offers the practitioner a number of choices. All commonly used circuits accomplish their goals more or less equivalently, but the simple act of increasing fresh gas flow, for example, may markedly increase the work of breathing. Therefore it is vital that the anesthesiologist understand the functional characteristics of each circuit.
Classifications of Breathing Circuits
A widely used nomenclature was developed that classified circuits as open, semiopen, semiclosed, or closed, according to whether a reservoir is used and whether rebreathing occurs. An open system has no reservoir and no rebreathing; a semiopen system has a reservoir but no rebreathing; a semiclosed system has a reservoir and partial rebreathing; and a closed system has a reservoir and complete rebreathing. Variations on this classification included the type of carbon dioxide absorber and unidirectional valves used.
Because of confusion with this traditional nomenclature, Hamilton recommended its abandonment in favor of both a description of the hardware (e.g., circle filter system, coaxial circuit, T-piece) and the gas flow rates being used. Identifying the circuits by eponym—such as Adelaid, Bain, Hafnia, Humphrey, Jackson-Rees, Lack, Magill, and Waters—did not help in understanding the function or application of the circuit. Almost all anesthesia machines are equipped with some form of a circle breathing circuit with the ability for carbon dioxide absorption during low-flow anesthesia and elimination through the pop-off valve during high-flow anesthesia. Because an understanding of how circuits work is essential, breathing circuits in this chapter are organized by method of carbon dioxide elimination. Methods for removal of carbon dioxide are discussed.
Chemical Absorption of Carbon Dioxide
Semiclosed and closed systems (i.e., circle and to-and-fro) rely on chemical absorption of carbon dioxide. Exhaled carbon dioxide is absorbed, and all other exhaled gases are rebreathed. The quantities of fresh oxygen and anesthetics equal those lost as a result of uptake, metabolism, and circuit leaks.
Dilution with Fresh Gas
Because of the intermittent nature of carbon dioxide excretion (during exhalation only) and the continuous inflow of fresh gas, the choice of inflow rate—as well as the locations of the inflow site, reservoir bag, and pop-off valves—contributes to the efficiency of carbon dioxide removal. When fresh gas flows are 1 to 1.5 times the minute volume (approximately 10 L/min in an adult), dilution alone is sufficient to remove carbon dioxide. Such systems then behave the same as a nonrebreathing system.
Use of Valves to Separate Exhaled Gases from Inhaled Gases
Systems that use nonrebreathing valves are examples of this method of carbon dioxide removal. A circuit that by virtue of high flows behaves as if it were nonrebreathing is not considered a nonrebreathing circuit in this analysis.
Use of Open-Drop Ether or a T-Piece Without a Reservoir to Release Exhaled Carbon Dioxide into the Atmosphere
Although similar to the second method above, systems that used open-drop ether or a T-piece without a reservoir were not truly breathing circuits. The T-pieces with an expiratory reservoir rely on dilution of carbon dioxide by both fresh gas and room air for its removal; these have been included in semiclosed circuits below.
Components of A Breathing Circuit
The circuits described above have many features in common; they connect to the patient’s airway through a face mask, laryngeal mask, or tracheal tube adapted to the breathing circuit through a Y-piece or elbow. The system may include valves to permit directional gas flow, and a reservoir bag is almost always present, which can be used to manually force gas into the lungs. Fresh gas must be supplied to the circuit, and excessive gas must be allowed to escape. In some, carbon dioxide is absorbed in a chemical filter. A variety of ancillary devices may also be present, such as humidifiers, spirometers, pressure gauges, filters, gas analyzers, PEEP devices, waste gas scavengers, and mixing and circulating devices.
Connection of the Patient to the Breathing Circuit
Either an anesthesia mask, supraglottic device, or a tracheal tube connects the circuit to the patient. Masks are made from rubber or clear plastic to make secretions or vomitus visible ( Fig. 4-1 ). Most have an inflatable or inflated cuff, a pneumatic cushion that seals to the face. Masks are available in a variety of sizes and styles to accommodate the wide variety of facial contours. For example, a prominent nasal bridge may prevent a tight fit if the mask’s cuff is flat at that point. A prominent chin (mentum) with sunken alveolar ridge causes a leak at the corner of the mouth, and the volume of the mask contributes to apparatus dead space. The mask should fit between the interpupillary line over the nose and in the groove between the mental process and the alveolar ridge ( Fig. 4-2 ). The average length of this area is 85 to 90 mm in adults. The newest disposable plastic masks are available in a wide range of sizes, intended to fit the faces of small children and large adults equally well. Choosing from a selection of mask sizes and styles is more rational than a “one size fits all” approach because a poorly fitting mask can result in trauma to the patient. This is especially true when the mask must be positioned above the eyebrows because it can cause pressure on, and possibly damage to, the optic and supraorbital nerves. Masks often have a set of prongs for attachment to a rubber mask holder or head strap; however, if pulled too tight, this mask holder may obstruct the airway. Masks connect to the Y-piece or elbow via a 22-mm (⅞-inch) female connection.


Breathing Tubing
The tubing used in breathing circuits typically is approximately 1 meter in length, has a large bore (22 mm) to minimize resistance to gas flow, and has corrugations or spiral reinforcement to permit flexibility without kinking. The internal volume is 400 to 500 mL/m of length. Although these tubes were formerly made of conductive rubber, disposable plastic tubing has almost completely replaced rubber. Electrical conductivity is no longer necessary when breathing tubing is used with nonflammable agents. The advantage of plastic is that it is lightweight; however, it is not biodegradable and thus is disposable by design although not by use. Plastic tubing for a breathing circuit is supplied sterile despite the lack of convincing epidemiologic data to support the necessity of sterile tubing. On occasion, it is necessary to pass a breathing circuit on to the sterile surgical field (e.g., during an ex utero intrapartum treatment procedure). By convention, the ends of the tubing are 22 mm in internal diameter (ID) and are identical in design. Tubing should be inspected before use because manufacturing errors can result in obstruction of the lumen. Compliance of the tubing varies from nearly 0 to more than 5 mL/m/mm Hg of applied pressure, and plastic tubing has lower values than rubber ( Table 4-1 ). Apparent distensibility is even greater because compression of gas under pressure, to the order of 3% of the volume, occurs at typical inflation pressures. Inflation of a patient’s lungs to 20 cm H 2 O peak inspiratory pressure compresses 30 to 150 mL of gas in the tubing. This volume is not delivered to the patient’s lungs, but some fraction of it may be measured by a spirometer within the circuit, adding a form of apparatus dead space to the system. The exact fraction depends on where the spirometer is placed in the circuit with respect to the unidirectional valves.
Circuit Pressure (cm H 2 O) | Volume (mL) | |
---|---|---|
Conductive Rubber | Disposable Plastic | |
10 | 80 | 30 |
15 | 130 | 100 |
20 | 210 | 190 |
25 | 290 | 320 |
Resistance to gas flow in standard, corrugated breathing tubes is exceedingly small—less than 1 cm H 2 O/L/min of flow. When it is desirable to have the anesthesia machine at some distance from the patient’s head, several tubes may be connected in series with connectors 22 mm (⅞ inch) in outside diameter (OD). Alternatively, extra-long tubing is available, including tubing that can be compressed to 200 mL of volume in approximately 50 cm of length or that can be stretched to nearly 2 m with an 800-mL volume. These “concertina” extensions do not increase the resistance of the system by any appreciable amount and affect the apparatus dead space only by their compliant volume ( Fig. 4-3 ).

The pattern of gas flow through the circuit is almost always turbulent because of the corrugations in the tubing, which promote both radial mixing and longitudinal mixing. In documenting performance of one circuit, Spoerel demonstrated complete mixing of dead space and alveolar gas after gas had passed through 1 m of such tubing. A change in gas composition at one end, such as when the delivered gas is altered at the anesthesia machine, completes a change in the inspired concentration at the patient connection within two to three breaths. The change in inspired concentration is nearly exactly the change in delivered concentration when high fresh gas flows are used (≥10 L/min). The change decreases to nearly imperceptible as inflow is decreased toward that of closed systems.
Lengths of breathing tubing are sometimes used to connect ventilators to the bag mount and to connect to scavenging devices. Optimally, either a 19- or 30-mm diameter ends on the scavenger mounts prevent inappropriate connections. Tubing of smaller diameter is made for use in circle systems designed specifically for infants and children, and their resistance to gas flow is insignificantly increased. With less compression volume, measured ventilation is more accurate.
Reusable rubber tubing is connected to the mask or tube by a separate Y-piece. Disposable sets often incorporate a Y that may or may not be detachable. Such a Y may be rigid, and it may incorporate an angle elbow or a pair of swivel joints. Although the swivel joints are convenient, they offer a greater chance of leaking; most connectors have negligible leakage, but those with swivels are twice as likely to leak. Any circuit should be tested before use by determining the oxygen inflow required to maintain 30cm H 2 O of pressure in the circuit (see also Chapter 32 ).
Unidirectional Valves
Unidirectional valves are incorporated into a breathing circuit to direct respiratory gas flow. They are commonly disks on knife edges or rubber flaps or sleeves. The essential characteristics of respiratory valves in breathing circuits are low resistance and high competence. The valves must open widely with little pressure and must close rapidly and completely with essentially no backflow.
Circle and nonrebreathing systems use two nearly identical valves: the inspiratory valve opens on inspiration and closes on expiration, preventing backflow of exhaled gas in the inspiratory limb. The expiratory valve works in a reciprocal fashion to prevent rebreathing. These valves can be mounted anywhere within the inspiratory and expiratory limbs of the circuit. The only critical feature of their location is that one must be placed between the patient and the reservoir bag in each limb. Properly positioned and functioning, they prevent any part of the circle system from contributing to apparatus dead space. Thus the only apparatus dead space in such a circuit is the distal limb of the Y-connector and any tube or mask between it and the patient’s airway. The respiratory valves on most modern anesthesia machines are located near, or incorporated into, the carbon dioxide absorber canister casing along with a fresh gas inflow site and excess gas (pop-off) valve. In the past, unidirectional valves have been incorporated into the housing of the Y-piece to decrease the apparatus dead space effect of compliance volume, but they have fallen into disfavor because of the weight they add to the mask. More importantly, they cause an obstruction to respiration if they are accidentally incorporated backward to the conventional valves in the circle. When valved Y-pieces were used, it was recommended that circle system valves be removed. Failure to reinsert the circle system valves when a normal nonvalved Y-piece was used has caused needless complications.
The common valves in anesthetic circuits are dome valves consisting of a circular knife edge occluded by a very light disk of slightly larger diameter ( Fig. 4-4 ). The disk lifts off the knife edge when flow is initiated by the patient’s inspiratory effort, when positive pressure is applied to the reservoir bag, or when the ventilator bellows empties. The disk is contained either by a small cage or by the dome itself. It must be hydrophobic so that water condensation does not cause it to stick to the knife edge and thereby increase the resistance to opening. Most modern disks are made of hydrophobic plastic and are light and thin. When properly functioning, the disk in a unidirectional valve can be lifted with a circuit pressure of 0.31 cm H 2 O or less. Most unidirectional valves are mounted vertically, with the disk oriented horizontally, so that it will fall properly into the closed position and seal the circuit from backflow. The valve disks also can be oriented vertically, as on the absorber block of the Datex-Ohmeda ADU workstation (GE Healthcare, Waukesha, WI; see Fig. 4-4 , D). Failure to seal converts a large volume of the circuit into apparatus dead space, resulting in rebreathing. The top of the valve is covered by a removable clear plastic dome so that the disk can be easily seen and periodically cleaned or replaced.


A nonrebreathing system requires two appropriately placed one-way respiratory valves ( Fig. 4-5 ). Nonrebreathing valves permit the patient to inspire fresh gas from a reservoir and exhale alveolar gas into the room or into a scavenger. Such valves usually consist of a pair of leaflets in the same housing: one opens during inspiration, the other opens during expiration. The early nonrebreathing valve designs, such as the Digby-Leigh or Steven-Slater, required the anesthesiologist to occlude the expiratory valve with a finger if assisted or controlled ventilation was needed ( Fig. 4-5 , A ). Modern designs that use springs, magnets, or flaps automatically close the expiratory valve when respiration is controlled. Other designs use the pressure difference across the inspiratory valve to inflate a mushroom-shaped balloon (Frumin) valve ( Fig. 4-5 , B ), or to depress a dome-shaped cover on the expiratory (Fink) valve. Resistance is negligible in both designs, but the Frumin valve has the marked advantage of collapsing if the inspiratory supply is inadequate, permitting inspiration of room air. The Frumin valve also is lighter and more compact than the others. Some nonrebreathing valves are position sensitive and must be vertically oriented to function properly. Those that use flexible rubber leaflets or collapsible rubber tubing to provide the sealing function are not positional. Most nonrebreathing valves connect to masks and/or tracheal tubes, but a valve can be built into a mask.

Self-inflating resuscitators for air or air-oxygen mixtures use similar pairs of valves to control gas flow. The Ruben valve has an expiratory bobbin-shaped structure that, when open, occludes the inspiratory limb ( Fig. 4-6 ). Anesthetic vapors and secretions tend to expand this bobbin slightly, causing it to jam. Such resuscitator valves should not be used in anesthesia, nor should they be used for transporting patients who are still exhaling anesthetic agents.

Breathing Bags
Breathing bags, also known as reservoir bags or counterlungs, have three principal functions: 1) they serve as a reservoir for anesthetic gases or oxygen, from which the patient can inspire; 2) they provide the means for a visual assessment of the existence and rough estimate of the volume of ventilation; and 3) they serve as a means for manual ventilation. A reservoir function is necessary because anesthesia machines cannot provide the peak inspiratory gas flow needed during normal spontaneous inspiration. Although the respiratory minute volume of an anesthetized adult is rarely more than 12 L/min, the peak inspiratory flow rate may reach 50 L/min, with 20 L/min not uncommon. For example, assume a patient is breathing at a rate of 20 breaths/min with a tidal volume of 500 mL and a minute volume of 10 L/min. If the inspiratory to expiratory ratio (I:E) is 1:2, each breath takes 1 second for inspiration and 2 seconds for exhalation. The tidal volume of 500 mL inspired in 1 second is an average inspiratory flow (volume per unit time) of 500 mL/sec or 30 L/min. This is many times greater than the commonly used fresh gas flows. The peak flow in mid-inspiration may be 30% to 40% higher.
Assessment of the presence and volume of spontaneous ventilation is affected by the fresh gas flow. In low-flow techniques, virtually all the gas inhaled by the patient comes from the reservoir bag, and its excursion thus reflects tidal volume. If the fresh gas inflow rate from the machine exceeds 10 L/min, most of the gas inhaled by the patient comes from the fresh gas supply, and the reservoir bag shows little excursion. In a spontaneously breathing patient with a circuit gas inflow rate of 6 L/min, nearly half the tidal volume comes from the fresh gas inflow, halving the apparent tidal volume as indicated by movement of the bag.
Reservoir bags for anesthesia machines usually are ellipsoid so they can be easily grasped with one hand. They are made of nonslippery plastic or latex in sizes from 0.5 to 6 L. To improve grip, some have an hourglass shape or a textured surface; nonlatex bags are available for use with patients who have a latex sensitivity. The optimally sized bag can hold a volume that exceeds the patient’s inspiratory capacity; that is, a spontaneous deep breath should not empty the bag. For most adults, a 3-L bag meets these requirements and is easy to grasp. Bags with a nipple at the bottom for use as an alternate pop-off site are available but are rarely used.
In circle systems, the breathing bag usually is mounted at or near the carbon dioxide absorbent canister via a T-shaped fitting, usually near the pop-off valve. The bag also may be placed at the end of a length of corrugated tubing leading from the T-connector to provide some freedom of movement for the anesthesiologist. The pressure-volume characteristics of overinflated bags become important if the pop-off valve is accidentally left in the closed position and gas inflow continues ( Fig. 4-7 ). Rubber bags become pressure limiting with maximum pressures of 40 to 50 cm H 2 O, although prestretching may favorably lower the maximum distending pressure. Disposable bags may reach twice the pressure of rubber bags and then rupture abruptly.

Gas Inflow and Pop-off Valves
Gases are delivered from the anesthesia machine common gas outlet to the circuit via thick-walled tubing connected to a nipple incorporated into the circuit. In circle systems this gas inflow nipple is incorporated with the inspiratory unidirectional valve or the carbon dioxide–absorbent canister housing. The preferred fresh gas inflow site is between the carbon dioxide absorber and the inspiratory valve. The location for other circuits depends on whether breathing is spontaneous, assisted, or controlled because the type of breathing influences the efficiency of carbon dioxide elimination.
Pop-off valves—also known as overflow, outflow, relief, spill, and adjustable pressure-limiting (APL) valves—permit gas to leave the circuit, matching the excess to the inflow of fresh gas. The efficiency of an APL valve is related in part to the placement of the fresh gas inflow. There are many different designs, but most are constructed like a dome valve loaded by a spring and screw cap ( Fig. 4-8 ). The valve should open at a pressure of less than 1 cm H 2 O. As the screw cap is tightened down, more and more gas pressure in the circuit is required to open it, permitting PEEP during spontaneous ventilation or pressure-limited controlled respiration. The number of clockwise turns from fully open to fully closed should be one or two: fewer turns make it difficult to set a desired circuit pressure accurately, whereas more make it tedious to use. The exhaust from any of the commonly used pop-off valves can be collected by scavenging system transfer tubing connected at this point.


The Datex-Ohmeda GMS absorber uses an APL valve similar in design to that shown in Figure 4-8 , which basically is a spring-loaded disk. When the spring is fully extended, it exerts a pressure of approximately 1 cm H 2 O on the disk to hold the valve closed. This is necessary because the waste gas scavenging interface is connected downstream of the APL valve and transfer tubing. If an active scavenging system is used—that is, if suction is applied to the interface—the negative pressure could potentially be applied to the patient circuit (see Chapter 5 ). To prevent this, the Ohmeda scavenger interface uses a negative-pressure relief (“pop-in”) valve that opens at a pressure of −0.25 cm H 2 O to allow room air to enter the interface. Thus the greatest negative pressure needed to open the APL valve (−0.25 cm) is less than the least spring tension needed to keep the valve closed (~1 cm H 2 O). This arrangement, with the use of an active scavenging system, protects against application of excess negative pressure to the breathing circuit. In the fully closed position, the maximum spring pressure applied to the Datex-Ohmeda APL valve disk is 75 cm H 2 O. Thus, in the manual/bag mode, the circuit pressure in an Datex-Ohmeda breathing system is limited to 75 cm H 2 O. Note that in the ventilator mode, the circuit pressure is limited by high pressure-limit settings on the Datex-Ohmeda ventilator (up to 100 cm H 2 O with the Datex-Ohmeda 7800 and 7900 ventilators; see Chapter 6 ).
In Dräger Medical (Telford, PA) anesthesia delivery systems, the design of the APL valve differs from those described above (see Fig. 4-8 , B ). This design uses a needle valve instead of a spring-loaded disk, and adjusting the knob varies the size of the opening between the needle valve and its seat, which in turn adjusts the amount of gas permitted to flow to the scavenger system. A check valve prevents gas from the scavenging system from entering the breathing system. With this design, the needle valve can be totally closed; it therefore does not function as a true pressure limiter.
Special types of pop-off valves permit spontaneous or assisted respiration without tedious adjustment. The simplest is the Steen valve ( Fig. 4-9 ), which essentially is two knife-edge valves of the dome type, one inverted over the other, that share a common disk. A relatively slow flow of gas during the latter part of exhalation, up to 10 L/min, lifts the valve disk at one side only so that the exhaled gas escapes around the disk. An abrupt increase in pressure lifts the valve vertically, seals it against the upper knife edge, and closes the circuit so that no gas is lost. The Georgia valve adds a light spring loading to the same design, which increases the range of gas flows it can exhaust; this is necessary for use with mechanical ventilators. Most current anesthesia ventilators have such an automatic pop-off valve built in so that gas is exhausted only at end exhalation (see also Chapter 6 ).

Carbon Dioxide Absorption
In partial rebreathing and nonrebreathing systems, carbon dioxide is vented to room air. When a closed system is used, however, the exhaled carbon dioxide must be otherwise removed. Carbon dioxide in the presence of water is hydrated to form carbonic acid. When carbonic acid reacts with a metal hydroxide, the reaction is one of neutralization that results in the formation of water and a metal bicarbonate or carbonate and the generation of heat. This reaction is used in anesthesia for carbon dioxide absorption. In the reactions shown below, only the molecular forms of the reactants are written. The reactions actually proceed by initial ionization in the thin film of water at the surfaces of the absorbent. In soda lime:
CO 2 + H 2 O → H 2 CO 3
H 2 CO 3 + 2 NaOH → Na 2 CO 3 + 2 H 2 O
H 2 CO 3 + 2 KOH → K 2 CO 3 + 2 H 2 O
Na 2 CO 3 + Ca ( OH ) 2 → 2 NaOH + CaCO 3
( or K 2 CO 3 ) ( or 2 KOH )
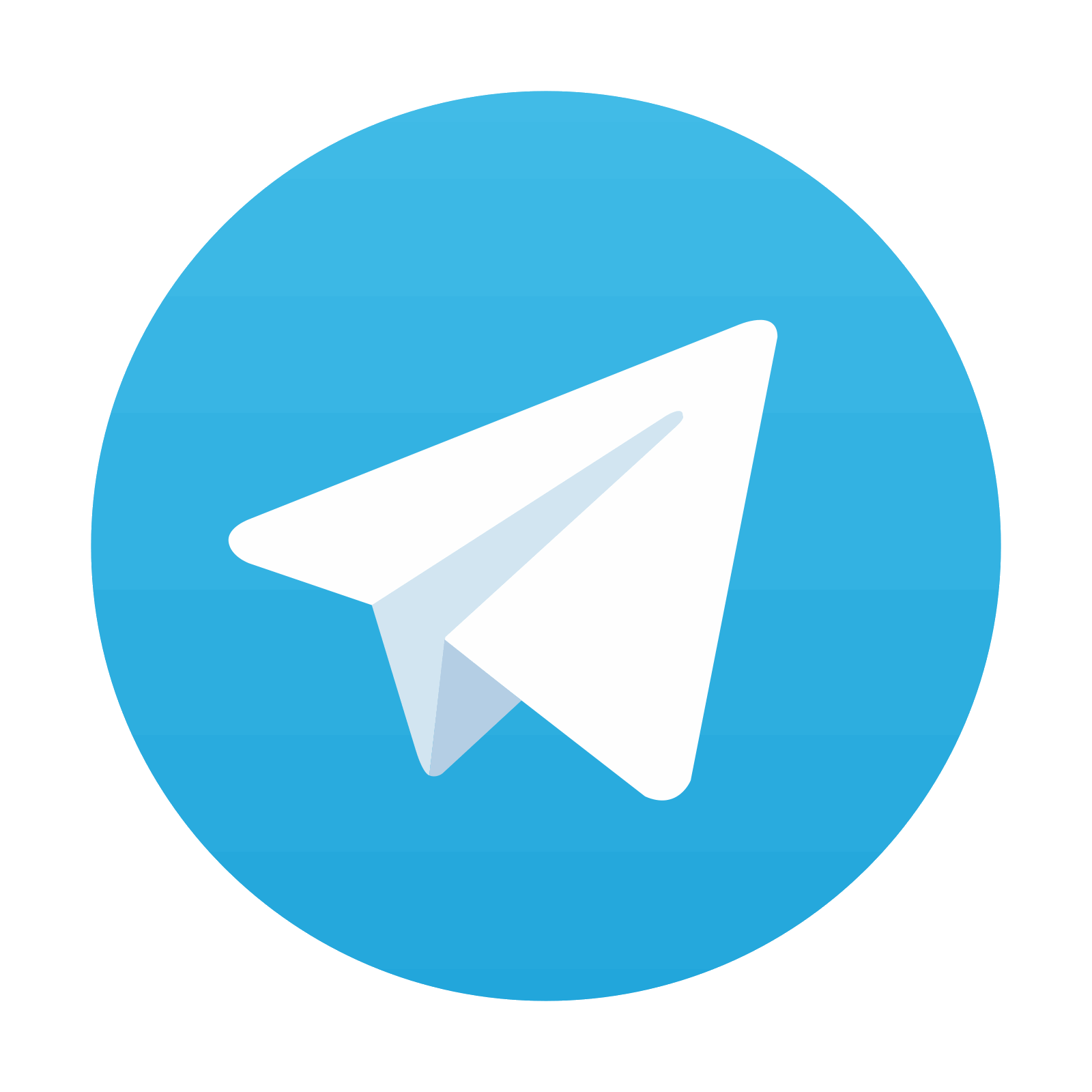
Stay updated, free articles. Join our Telegram channel
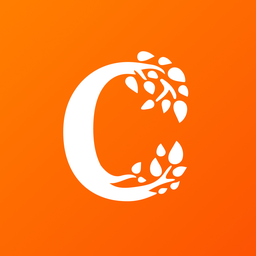
Full access? Get Clinical Tree
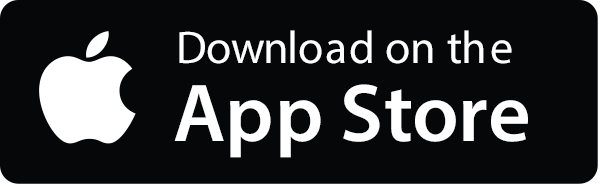
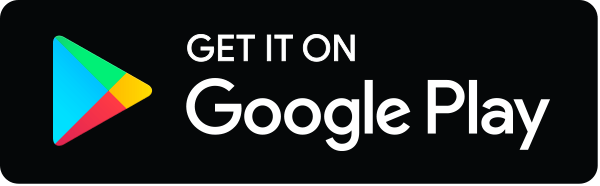