Pediatric Anesthesia
Jerrold Lerman
Key Points






children are linked to the severity of intermittent nocturnal desaturation (threshold is oxygen saturation (SaO2) <85%) as they upregulate genes responsible for opioid sensitivity. Emerging evidence suggests that sleep apnea in obese child is complicated by a systemic inflammatory response, resulting in less favorable outcomes after adenotonsillar surgery.




Related Matter
Single Breath Inhalational Induction
Portions of this chapter may appear in Lerman J, Sampathi V, Watt S. Induction, maintenance, and emergence from anesthesia. In: Andropoulos D, Gregory G, eds. Pediatric Anesthesia. 2012:330–360; Chapter 15. Wiley-Blackwell, Oxford, UK
Anatomy and Physiology

Understanding the anatomical differences between the infant and adult upper airways is key to managing the infant’s airway safely. Table 42-1 summarizes these differences. As a result of these anatomical features, the head in the child is naturally in the “sniffing” or flexed position. The large tongue/mouth ratio presents difficulty if the mouth is closed during mask ventilation, particularly with the narrowed nares. Hence, mask ventilation requires particular skill to avoid airway obstruction. Ensuring safe mask anesthesia and a patent airway requires proper application of the “jaw thrust” as described below while avoiding pressure on the soft tissues in the submental triangle.
The most common airway problem in infants and young children is upper airway obstruction due to laryngomalacia. In this condition, the supraglottic structures converge on the glottic opening during inspiration preventing most, if not all, air entry through the glottis. The net effect is airway obstruction that is characterized by suprasternal and supraclavicular retractions, paradoxical collapse of the chest wall and/or sternum, and exaggerated diaphragmatic excursions especially if the child becomes distressed. Positive airway pressure usually resolves the acute condition. This condition resolves itself as the children grow and the oropharyngeal structures develop although in some instances surgery may be required.
A number of airway anomalies may present problems during anesthesia. Some anomalies improve with age (e.g., Pierre Robin sequence defined as micrognathia, airway distress in the first 24 hours after birth, and glossoptosis) generally becoming easy laryngoscopies around 2 years of age whereas others (such
as Treacher Collins syndrome, mucopolysaccharidoses) become progressively more difficult mask anesthetics and laryngoscopies with age. Several disorders present primarily with difficult mask anesthetics and ventilation while tracheal intubation is usually straightforward (e.g., Crouzon’s and Apert’s diseases and Down syndrome). Most airway problems in children present difficulties with tracheal intubation. It is rare to face a “cannot ventilate, cannot intubate” airway in a child. Nonetheless, it is essential to identify a child’s syndrome and determine whether the airway is involved.
as Treacher Collins syndrome, mucopolysaccharidoses) become progressively more difficult mask anesthetics and laryngoscopies with age. Several disorders present primarily with difficult mask anesthetics and ventilation while tracheal intubation is usually straightforward (e.g., Crouzon’s and Apert’s diseases and Down syndrome). Most airway problems in children present difficulties with tracheal intubation. It is rare to face a “cannot ventilate, cannot intubate” airway in a child. Nonetheless, it is essential to identify a child’s syndrome and determine whether the airway is involved.
Table 42-1. Anatomical Features of the Upper Airway in Infants Compared with Adults | ||||||||
---|---|---|---|---|---|---|---|---|
|
The cricoid ring represents the only solid cartilaginous structure in the upper airway because it is covered with pseudostratified columnar epithelium. This epithelium is subject to swelling if irritated, swelling into the lumen and reducing the radius of the airway. Because airflow in the upper airway is turbulent (Reynolds number >4,000), pressure decrease across the cricoid ring increases with changes in the radius to the fifth power. Hence, a 50% reduction in the radius of the cricoid ring increases the pressure drop by 32-fold. This increases the work of breathing, which cannot be sustained for long, resulting in respiratory failure.
The short trachea in the infant and child facilitates accidental endobronchial intubation. Careful assessment of the position of the tracheal tube in the airway is crucial to avoid this problem. Persistent hemoglobin desaturation (SaO2 <85%) should suggest an endobronchial intubation.
The increased alveolar ventilation to functional residual capacity in the child increases the risk of hemoglobin desaturation. The increased alveolar ventilation arises from the increased oxygen consumption per kilogram required by the child compared with the adult. This fact along with the increased compliance of the rib cage (due to both anatomical and physiologic features), reduced compliance of the lungs (due to the relative lack of elastin in the infant), and reduced percent of type 1 fibers (slow-twitch, high oxidative muscle fibers) in the diaphragm predisposes the basal segments of the lungs to atelectasis under the weight of the abdomen. Together, these factors predispose the infant to rapid desaturation and respiratory failure when faced with respiratory difficulties. Additional details of the physiology of the pulmonary system may be found in Chapter 41.
Cardiovascular
Once the neonatal heart completes the transition to postnatal life, the changes in the cardiovascular system are less dramatic. In the early years, the heart has limited ability to increase stroke volume, rendering cardiac output more dependent on heart rate than in the adult. A corollary of this relationship is that hypotension in the child with a normal or increased heart rate is due to hypovolemia and should be treated with volume expansion rather than vasopressors (except in children with congenital heart disease). Systemic vascular tone is low in children up to 8 years of age, as evidenced by the lack of change in blood pressure when caudal/epidural blocks are administered.
Both heart rate and blood pressure increase with increasing age in childhood (see Table 42-2)1 and these provide a framework from which the definitions of bradycardia and hypotension were developed.
Central Nervous System
Physiology
Oxygen consumption in the brain of children (5.5 mL/100 g/min) is 50% greater than that in adults (3.5 mL/100 g/min).2 As a result, cerebral blood flow differs substantially from adults. In children 6 months to 3 years of age, the cerebral blood flow is 50% to 70% greater than in adults (50 mL/min/100 g vs. 70 to 110 mL/min/100 g). More cerebral blood flow is directed to the gray matter in children, reaching a distribution similar to that in adults by adolescence.
Table 42-2. Normal Range of Resting Heart Rates and Blood Pressure in Children | |||||||||||||||||||||||||||
---|---|---|---|---|---|---|---|---|---|---|---|---|---|---|---|---|---|---|---|---|---|---|---|---|---|---|---|
|
Apoptosis

Whether the rodent and nonhuman primate evidence of anesthetic-induced neurotoxicity is applicable to humans remains hotly debated. Since most anesthetics in young children last less than 3 hours, these data may have limited external validity, at least in terms of humans. Second, the doses of intravenous anesthetics and sedatives in rodents and primates are up to 10-fold greater than in humans4; such large doses may explain, in part, the neurocognitive dysfunction observed after intravenous anesthetics. Third, studies in humans who received anesthesia at a young age suggested that cognitive disability in those who received anesthesia before the age of 3 years may be more prevalent than in those who did not.3 However, most of those studies were seriously flawed in terms of their design (retrospective), limited external validity (no pulse oximetry or capnography), different anesthetics (halothane), nonstandardized metrics (learning disability tests were not applied equally to all children), and confounding variables (complex pregnancy, drugs such as magnesium) that were not standardized. However, a large cohort of identical twins who were discordant for general anesthesia at <3 years of age in one study, when tested for intellectual aptitude 10 years later, were similar.5 Prospective studies that are currently underway may clarify this issue. It is intriguing to know
that rodents that have received a cerebral insult from ischemia, trauma or sevoflurane anesthesia have less cognitive dysfunction if they are exercised after the insult.6,6a
that rodents that have received a cerebral insult from ischemia, trauma or sevoflurane anesthesia have less cognitive dysfunction if they are exercised after the insult.6,6a

Developmental Pharmacology
Understanding the pharmacology of medications in children is a large undertaking and a complex subject that is briefly addressed below.
For medications to be effective, they must reach their effect site. The steps involved in that process include absorption of the medication into the blood, transfer from the blood to the effect site, and termination of its action by redistribution, metabolism, and excretion.
The bioavailability of medications that are not administered intravenously depends on several factors including the route of administration, pKa, and solubility of the drug and local perfusion. Some medications such as midazolam are poorly absorbed from the stomach at clinical doses (15% bioavailability)7 but are well absorbed via the nares8 whereas others such as acetaminophen are well absorbed from the stomach but are poorly and erratically absorbed from the rectum.9 Age may have a substantial effect on the absorption of these drugs for example, gastric juice is closer to neutral pH (pH ∼6) at birth and does not reach adult levels of acidity until ∼3 years of age.10 Since drugs may be administered by any of these routes, it is critical to evaluate the bioavailability of the medication via each route to determine the appropriate dose required to achieve an adequate blood concentration. The route of administration also affects whether the drug undergoes first-pass metabolism through the liver. Rectal venous drainage from the superior hemorrhoidal veins drains into the portal venous system, whereas blood from the middle and inferior hemorrhoidal veins bypasses the liver flowing directly into the iliac veins and on to the heart. Hence medications that are administered rectally may undergo first-pass hepatic metabolism if they are absorbed via the superior rectal veins.
Once in the blood, intravenous medications partition between the protein-bound fraction and the free or active fraction. Two major proteins bind medications: Albumin and α1-acid glycoprotein. Both are synthesized in the liver. Albumin concentration is reduced in children with many diseases (cancer, nephropathy) and malnourishment. It binds acidic medications. α1-acid glycoprotein concentration is also reduced at birth but increases slowly with increasing age as well as during periods of stress.11 It binds basic compounds. Hence, the free fraction of medications such as lidocaine is greater in young infants than in older children because the concentration of α1-acid glycoprotein in the former is reduced.11 As the free fraction of medications increases, a greater dose will reach the effect site and exert physiologic or, in the case of an overdose, adverse events.
Termination of the action of most intravenous agents depends on their metabolism by the liver through phase 1 reactions (e.g., hydroxylation and oxidation) and/or phase 2 reactions (e.g., glucuronidation). The rates at which these enzyme systems mature vary widely among and within individuals depending on a host of factors. Apart from a few enzyme systems that hold importance in fetal life (e.g., CYP450 3A7),12 the activities of the vast majority of CYP450 enzyme systems (e.g., CYP450 3A4, 2E1, and 2D6) increase with age from birth, but at divergent rates (Fig. 42-1).12 Moreover, genetic polymorphisms of several families of enzymes (e.g., 2D6) may dramatically affect the activity of that enzyme system resulting in a wide range of activity from none to rapid and excessive (see Codeine below).13
Phase 1 reactions are responsible for the majority of drug metabolism in the liver acting via the cytochrome P450 enzyme system. Several enzymes are responsible for the metabolism of the vast majority of medications we administer: 3A4 (50% of medications), 2D6 (10% to 20% of medications),13 1A2, 2E1, and 2C9. These isozyme systems mature from birth, at which time most have limited activity (Fig. 42-1). Most of these enzyme systems mature to adult activity levels by 1 to 5 years of age. Phase 2
systems are also immature at birth, giving rise to concerns about bilirubin toxicity. However, these systems also mature quickly with age.
systems are also immature at birth, giving rise to concerns about bilirubin toxicity. However, these systems also mature quickly with age.
Table 42-3. Pharmacology of Inhaled Anesthetics | ||||||||||||||||||||||||||||||||||||||||||||||||||||||||||||||||||||||||||||||||||||||||||
---|---|---|---|---|---|---|---|---|---|---|---|---|---|---|---|---|---|---|---|---|---|---|---|---|---|---|---|---|---|---|---|---|---|---|---|---|---|---|---|---|---|---|---|---|---|---|---|---|---|---|---|---|---|---|---|---|---|---|---|---|---|---|---|---|---|---|---|---|---|---|---|---|---|---|---|---|---|---|---|---|---|---|---|---|---|---|---|---|---|---|
|
Termination of the action of many medications in anesthesia depends on either redistribution of the active compound from the effect site to other vessel-rich organs (see Inhalational section) or muscle, or metabolism in the liver and excretion or direct excretion by the kidneys. Elimination of the metabolic by-products and residual active parent compounds depends on renal perfusion and elimination. The glomerular filtration rate is markedly reduced in the neonate but matures throughout childhood reaching adult rates by 5 to 15 years of age.14

The widespread appeal of the current inhalational anesthetics may be attributed to their physicochemical properties (Table 42-3), which provide a rapid onset and offset of action, cardiorespiratory homeostasis, and limited metabolism and toxicity. Halothane has all but disappeared in North American pediatric anesthetic clinical practice, having been replaced by sevoflurane as the induction agent of choice in infants and children. Enflurane has been supplanted by its optical isomer, isoflurane. Desflurane offers the most favorable pharmacokinetic and in vivo metabolic characteristics in terms of its minimal blood and tissue solubilities and resistance to metabolism, although its use as an induction agent in children is proscribed because it irritates the upper airway. Most recently, the noble gas xenon has generated much interest as an anesthetic because it is safe for the environment, lacks cardiovascular toxicity, and has no serious toxicity either in vivo or in vitro. However, xenon is very expensive, its minimum alveolar concentration (MAC) is 70%, and it causes nausea and vomiting.
All of the currently used inhalational anesthetics are methyl ethyl ether compounds except for sevoflurane, which is a methyl isopropyl ether (Table 42-3).
Pharmacokinetics
The rate of increase in alveolar to inspired anesthetic partial pressures (fraction in the alveolus (FA) to the fraction in the inspired gas (FI), known as the washin ratio, FA/FI) for inhalational anesthetics depends on six factors. The first three determine the delivery of anesthetic to the lungs and the second three determine the removal of anesthetic from the lung. The washin ratio increases from 0 toward 1 in the shape of an exponential curve for all inhalational anesthetics. The order of washin of the anesthetics is inversely related to the solubility of the anesthetics in blood; that is, the smaller the solubility in blood, the more rapid the washin.18
Table 42-4. Factors Increasing the Washin of Inhalational Anesthetics in Children Compared with Adults | |
---|---|
|
The washin curve for inhalational anesthetics is characterized by the simple exponential equation

where k is a constant (k = 1/time constant (τ)) and t is time in minutes. τ is the ratio of the volume of the organ to the blood flow to that organ. The smaller the time constant, the more rapidly FA/FI equilibrates. In children, the time constants for most organs are less than those in adults and this explains in part, the rapid equilibration of halothane in children compared with adults (Fig. 42-2).
In the case of infants and children, four factors explain the more rapid washin of halothane compared with adults. These factors are listed in order of importance from the greatest to the least in Table 42-4.
The greater alveolar ventilation: (VA) functional residual capacity (FRC) ratio in infants and children compared with adults may be attributed to the greater metabolic rate and oxygen demand in children. Increases in alveolar ventilation speed the equilibration of inspired to alveolar anesthetic partial pressures.19 The net effect of a greater VA: FRC ratio is a reduction in the time constant, from 0.7 in adults to 0.2 in infants, which explains the speed of equilibration. Although a greater cardiac output should slow the rate of increase in FA/FI, it actually speeds the equilibration in neonates and infants. This paradoxical effect may be attributed to the greater cardiac output perfusing the vessel-rich group (VRG) (comprised of heart, brain, gastrointestinal organs, kidneys, and endocrine glands) in infants which comprises 18% of the body’s weight compared with 8% in adults. Hence, the greater cardiac output in infants speeds the equilibration of FA/FI in the VRG, which takes up most of the anesthetic during the first couple of hours of anesthesia. The third most important factor in the rapid washin of anesthetics in infants is their reduced tissue solubility compared with that in adults.15 The solubility of all inhalational anesthetics is reduced in tissues in infants compared with adults including the brain, muscle, and heart. Lastly, the reduced solubility of anesthetic in blood in infants speeds the washin of inhalational anesthetics in infants.17
To understand the relevance of differences in the tissue solubility among age groups, the τ is defined by

In the brain, the brain blood flow is 50 mL/100 g/min or 50 mL/100 mL/min brain. In adults, λbrain/blood is ∼2, whereas in the infant it is 1. Substituting these values into Eq. 2 yields 100 × 2 (or 1)/50 or 4 minutes in adults or 2 minutes in children. Since 4τ are required to achieve 98% equilibration of FA/FI, the partial pressure of brain reaches alveolar pressures by 16 minutes in adults and 8 minutes in children (excluding the time to equilibrate anesthetic throughout the anesthesia workstation [AWS] and lungs). Hence, we can explain, in part, the sudden and rapid responses to inhalational anesthetic in infants and children compared with adults.
In contrast to the more soluble anesthetics of the past, the current inhalational anesthetics are less soluble in both blood and tissues. Since changes in alveolar ventilation and cardiac output affect the washin of less soluble anesthetics to a lesser extent than more soluble anesthetics, the first two factors in Table 42-4 have attenuated effects on the washin of sevoflurane and desflurane in young children. Therefore, the washin in this age group may not be substantively greater than it is in adults. Furthermore, the solubilities of these agents in blood in infants are similar to those in adults16; the tissue solubilities in infants compared with adults have not been reported. Hence, we expect the washin of sevoflurane and desflurane in infants to be marginally more rapid than in adults.
Estimates of the effect site equilibration half-life (t1/2keo) for sevoflurane in adults and children have been reported using the bispectral index (BIS) to be 3.2 and 1.2 minutes, respectively.20,21
Two additional aspects of the pharmacokinetics of inhalational anesthetics merit consideration in infants and children. The first is the mode of ventilation. The washin of inhalational anesthetics increases rapidly during induction of anesthesia. In dogs, the washin of halothane was well tolerated during spontaneous respiration whether the inspired concentration was 0.4% or 4%.22 The FA/FI plateaued in all instances at 0.6 to 0.7. As the anesthetic depth increased, ventilation was reduced, decreasing the intake of anesthetic. When the depth of anesthesia was reduced, ventilation increased and the intake and depth of anesthesia increased. This is a negative feedback control loop. However, when ventilation was controlled, 85% and 100% of the dogs that received 4% and 6% inspired halothane concentrations, respectively, did not survive.22 Controlled ventilation is a positive feedback control loop where the delivery of inhalational anesthesia continues unabated without the negative feedback effect of respiratory depression. Ultimately, as cardiac output decreases, the uptake of anesthetic decreases and the partial pressure in the lungs increases steadily. These greater partial pressures in the lungs equilibrate with the reduced cardiac output, which circulates to the VRG to further depress them, ultimately resulting in a cardiac arrest. This study illustrates the benefits of the negative feedback control of spontaneous ventilation during inhalational anesthesia that protects against an anesthetic overdose and the risks associated with controlled ventilation. In the past, since more than one vaporizer could be used simultaneously during
controlled respiration, interlocking devices were developed to prevent an inadvertent anesthetic overdose when the children could not protect themselves during controlled ventilation.
controlled respiration, interlocking devices were developed to prevent an inadvertent anesthetic overdose when the children could not protect themselves during controlled ventilation.
The second issue relates to shunts and their effects on the uptake and distribution of anesthetics. Left to right shunts have limited effects on the uptake and distribution of inhalational anesthetics provided the cardiac output is maintained.23 However, right to left shunts present an entirely different and far more complex clinical problem. Whether these shunts are intrapulmonary or intracardiac or both, the washin of less soluble anesthetics are far more substantively affected in the presence of these shunts than more soluble anesthetics.23 That is, it is much more difficult to maintain an adequate depth of anesthesia with sevoflurane in infants with significant right to left shunts. To maintain anesthesia, supplemental intravenous anesthesia is often used. Understanding this differential effect of shunts on the washin of inhalational anesthetics is beyond the scope of this chapter, but the basis may be summarized by the differential effect of ventilation on the washin of less and more soluble anesthetics.23
The speed of washout of inhalational anesthetics follows an exponential decay, with the speed inversely parallel to the solubility of the anesthetics in blood. That is, the washout is more rapid for anesthetics that are less soluble: Desflurane > sevoflurane > isoflurane ≥ halothane.18 The only exception to this rule is the washout of halothane, which overlaps that of isoflurane in part, because the former is metabolized 15% to 25% in vivo (Table 42-3). Simulation demonstrated greater differences in recovery among the anesthetics after prolonged anesthesia and to >90% decrement in the anesthetic concentration.24
Substituting a less soluble anesthetic for a more soluble one has been proposed as a technique to accelerate the washout of anesthetics and recovery from anesthesia. However, switching from isoflurane to sevoflurane 30 minutes before the end of surgery did not speed the recovery in one report.25 More recently, charcoal filters have been used to adsorb anesthetics and, when combined with hypercapnic hyperventilation in adults, were shown to rapidly remove anesthetics and speed recovery.26,27
Pharmacodynamics
The MAC is that concentration of inhalational anesthetic to which 50% of the patients move in response to a skin incision. In children, the MAC is known to depend to a great extent on age. That is, as the fetus matures and reaches term, the MAC increases peaking in infants 1 to 6 months of age for halothane28,29 and isoflurane30,31 and then decreases steadily thereafter with increasing age (Fig. 42-3). In the case of sevoflurane, the MAC is 3.3% in neonates and 3.2% in infants 1 to 6 months of age.32 For children 6 months to 12 years, the MAC is constant at 2.4%.32 In the case of desflurane, the MAC increases throughout infancy peaking in infants 6 to 12 months of age and decreases thereafter with increasing age.33 The reason for the age-dependent differences in the MAC is unclear.
The MAC values of inhalational anesthetics in children have shown several other peculiarities. The MAC of halothane is 25% less in children with cognitive dysfunction, especially those taking antiseizure medications.34 The MAC of desflurane in adult redheads is 20% greater than in nonredheads35; the same response would be expected in children of similar genetic predisposition. The MAC values for halothane and isoflurane show simple additivity with N2O in adults and children; however, the MAC values for sevoflurane and desflurane in children are only reduced 20% to 25% in the presence of 60% N2O.32,36 The reason for this effect of sevoflurane and desflurane is unknown. The MAC also varies with the child’s temperature. In children 4 to 10 years of age, the MAC of isoflurane decreases 5%/°C decrease in temperature.37
In addition to determining the MAC responses to skin incision, the ED50 to a number of other maneuvers including insertion and removal of laryngeal mask airways (LMAs), tracheal extubation, and others have been determined.38
Respiration
When administered in the absence of surgical stimulation, all anesthetics depress respiration and minute ventilation in a dose-dependent manner, by decreasing tidal volume and increasing respiratory rate.39,40 Inhalational anesthetics relax intercostal muscles before the diaphragm, resulting primarily in diaphragmatic respiration. Respiratory rate increases during anesthesia, which offsets in part the reduced tidal volume. These effects are most pronounced with halothane; at concentrations ≥1.4 MAC, sevoflurane depresses respiration to a greater extent than halothane in adults,41 although the evidence is less clear in children.40 As the concentration of sevoflurane increases, respiratory rate also diminishes ultimately resulting in apnea. This effect is augmented in the presence of a midazolam premedication. This central effect is offset by manually assisting ventilation. In the case of desflurane, concentrations >1 MAC depress respiration in infants and children, an effect that exceeds the depression by other inhalational anesthetics.42
Inhalational anesthetics also depress the response to carbon dioxide and hypoxia in a dose-dependent manner.
Airway resistance increases during desflurane anesthesia,43 and decreases during sevoflurane anesthesia.43,44 Hence, in children with asthma, the former is best avoided and the latter is preferred. In both children and adults in the intensive care unit with refractory status asthmaticus, inhalational anesthetics have been effective.45,46
Upper airway responses to inhalational anesthesia (by mask) depend on both the concentration and the particular anesthetic administered. Halothane and sevoflurane do not trigger
these reflex responses39,47 whereas isoflurane and desflurane are most irritating to the airway, particularly at >1 MAC concentrations.48,49 The package insert for desflurane cautions against using it for inhalational inductions in children. The mechanism by which inhalational anesthetics trigger upper airway reflex responses remains unclear.
these reflex responses39,47 whereas isoflurane and desflurane are most irritating to the airway, particularly at >1 MAC concentrations.48,49 The package insert for desflurane cautions against using it for inhalational inductions in children. The mechanism by which inhalational anesthetics trigger upper airway reflex responses remains unclear.
When a tracheal tube is used, neither desflurane nor isoflurane triggers airway reflexes either during anesthesia or emergence.49 When an LMA is used, extubation during deep desflurane anesthesia may increase the incidence of airway reflex responses when compared with extubation awake after desflurane or isoflurane.50
Cardiovascular
Inhalational anesthetics depress the heart in a dose-dependent manner. Direct effects of these anesthetics depress the heart rate, contractility, and peripheral vascular tone. Halothane depresses heart rate the most of these anesthetics, often sensitizing the myocardium to catecholamines and inducing ventricular dysrhythmias. In the past, anticholinergics were commonly used to prevent bradycardia and arrhythmias in children given halothane; however, this practice is no longer necessary with the newer ether anesthetics that infrequently cause arrhythmias. In contrast, sevoflurane and the remaining ether anesthetics exert no substantial effect on the cardiac conduction system. Sevoflurane maintains or increases heart rate during induction of anesthesia in most instances32 likely due to withdrawal of vagal tone,51 although on occasion nodal bradycardia has been reported during sevoflurane. Desflurane and isoflurane tend to increase the heart rate. Desflurane to a greater extent than isoflurane causes a sympathetic discharge when the inspired concentration increases rapidly and in a stepwise manner, without pretreatment with opioids52; similar responses in children have not been forthcoming. This effect is mediated through the right and left lungs.53 Sevoflurane and the other inhalational anesthetics prolong the QT interval, but sevoflurane does not increase the dispersion of repolarization, making arrhythmias very rare.54 Arrhythmias can occur in children with congenital long QT interval particularly during emergence from anesthesia.55
Halothane depresses myocardial contractility to the greatest extent; sevoflurane and the remaining ether anesthetics exert a much more attenuated effect in children. Hemodynamic responses to 1 × MAC of ether anesthetics suggest that they all decrease systolic blood pressure ∼20% to 30% from awake values, an effect that is usually reversed with surgical stimulation.30,32,33 Sevoflurane and halothane decrease cardiac index in children similarly, ∼10% at 1 × MAC and 20% to 30% at 2 × MAC, although sevoflurane may depress cardiac index in infants less than after halothane.56,57
Early studies showed the dependency of cardiac output in young children on heart rate. An increase in heart rate reversed halothane-dependent decreases in cardiac output.58 More recent evidence suggests that children can increase their stroke volume if needed to augment their cardiac output. Peripheral vascular resistance is very low in children as evidenced by the absence of a change in blood pressure when a caudal/epidural block is administered.
Sevoflurane is superior to halothane in children with congenital heart disease. It causes fewer arrhythmias and less hypotension than halothane.59
Most anesthetic medications prolonged the QT interval, but when the QT interval exceeds 500 milliseconds, the QT interval is considered prolonged.60 When a prolonged QT interval occurs in the presence of a dispersion of repolarization, there is a substantial risk for the occurrence of torsades de pointes. In the presence of drugs that prolong the QT interval (e.g., most anesthetics), the risk of torsades de pointes is increased in the presence of congenital long QT interval (Romano–Ward and Jervell and Lange-Nielson syndrome), nonanesthetic medications, several medical conditions (hypomagnesemia, hypokalemia, hypocalcemia, cardiac disease, hypothyroidism), bradycardia, and the female gender.60,61
Central Nervous System
All inhalational anesthetics decrease cerebral vascular resistance and cerebral metabolic rate for oxygen. The decrease in vascular resistance increases cerebral blood flow in the following order: Halothane > desflurane > isoflurane > sevoflurane.2 Sevoflurane and isoflurane decrease oxygen consumption to greater extents than halothane. Hence, the most favorable ratio of cerebral blood flow to oxygen consumption follows the reverse order: Sevoflurane > isoflurane > desflurane > halothane.
The effects of changes in blood pressure as well as carbon dioxide and oxygen tensions on cerebral blood flow during anesthesia in children have not been fully elucidated. Autoregulation of cerebral blood flow in children of all ages is similar to that in adults, although it occurs at greater blood flow rates.2 The lower limit of autoregulation in children of all ages appears to be similar, 60 mm Hg mean arterial pressure. All inhalational anesthetics impair autoregulation, although sevoflurane does not impair autoregulation in children ≤1.5 MAC.2 As in adults, hyperventilation restores autoregulation with isoflurane and sevoflurane. Cerebrovasodilatation to increasing carbon dioxide tension in children appears to be maintained up to ∼50 mm Hg, but beyond that level maximum vasodilatation prevents any further response. Changes in cerebral blood flow in response to changes in pCO2 during isoflurane anesthesia are greater than during sevoflurane. Hence, hyperventilation may be more effective in attenuating increased intracranial pressure during isoflurane anesthesia than during sevoflurane.
The electroencephalogram during sevoflurane is characterized by sharp, slow waves in the lower frequency range. This pattern differs substantially from that of the other anesthetics, resulting in some difficulties in interpreting BIS readings in children. That is, BIS readings are imprecise in children <5 years. They increase at sevoflurane concentrations >3%, are associated with marked variability, and are generally less than those of halothane but similar to isoflurane and desflurane at equipotent concentrations and reduced in cognitively challenged children by ∼25%.62,63,64,65,66 Myoclonic movement as well as EEG spike and wave activity (epileptiform) has been reported in a small number of children during inhalational inductions with sevoflurane, at concentrations 5% to 7%.67 In patients with seizures, epileptiform EEG activity was suggested in 58% of those anesthetized with 1 to 2 MAC sevoflurane and 25% of those with 1 to 2 MAC isoflurane, with the addition of hyperventilation actually reducing the epileptiform activity.68 With sevoflurane, these occurred not only in several patients with a history of seizures, but also in the presence of hyperventilation. Indeed, at sevoflurane concentrations approaching 8% during induction of anesthesia, ventilation should be assisted, not controlled with hyperventilation, if apnea occurs.
Renal
Inhalational anesthetics do not exert substantive effects on the kidneys in children except through their metabolism: The kidney is a site of degradation of inhalational anesthetics. Ether anesthetics, most notably methoxyflurane and to a lesser extent
sevoflurane, are susceptible to CYP450 2E1 metabolism69 releasing inorganic fluoride in similar concentrations, although only the former is known to be nephrotoxic. In part, this has been attributed to the presence of CYP450 2E1 in the kidneys and to the local release of inorganic fluoride, which is toxic to the renal tubules and in the case of methoxyflurane, caused high output renal failure and its withdrawal from the market.70 The difference in nephrotoxicity between the two anesthetics has been attributed to two factors: The first is that the affinity of methoxyflurane for 2E1 is several folds greater than that for sevoflurane. The second is that methoxyflurane is the only anesthetic to undergo O-demethylation, which produces dichloroacetic acid, a putative cofactor in the pathogenesis of anesthetic-induced nephrotoxicity.71
sevoflurane, are susceptible to CYP450 2E1 metabolism69 releasing inorganic fluoride in similar concentrations, although only the former is known to be nephrotoxic. In part, this has been attributed to the presence of CYP450 2E1 in the kidneys and to the local release of inorganic fluoride, which is toxic to the renal tubules and in the case of methoxyflurane, caused high output renal failure and its withdrawal from the market.70 The difference in nephrotoxicity between the two anesthetics has been attributed to two factors: The first is that the affinity of methoxyflurane for 2E1 is several folds greater than that for sevoflurane. The second is that methoxyflurane is the only anesthetic to undergo O-demethylation, which produces dichloroacetic acid, a putative cofactor in the pathogenesis of anesthetic-induced nephrotoxicity.71
Isoflurane and desflurane are metabolized to small extents. The risk of nephrotoxicity from inorganic fluoride from either anesthetic is remote. Indeed, very small inorganic fluoride concentrations (mean value 11 μM) have been detected after 131 MAC-hours isoflurane in children.72
Hepatic
There are few data regarding the effects of inhalational anesthetics on hepatic function in children. However, isolated cases of hepatic dysfunction in children have been reported after every inhalational anesthetic.73,74 Most children who develop hepatic dysfunction recover without proceeding to liver transplantation. In the case of halothane hepatitis, serologic markers in the form of antibodies to hepatic cell membrane antigens have been detected.75 Similar immunologic markers have been detected after isoflurane and desflurane, although none have been identified for sevoflurane to date. Although it has been suggested that repeated anesthetics with halothane cause hepatitis, this author asserts there is insufficient evidence to avoid repeated inhalational anesthetics in children.
In Vitro Metabolism
Degradation of inhalational anesthetics in the presence of carbon dioxide absorbents has been the subject of intense research and concern in both adults and children.
Sevoflurane may be degraded via the Cannizzaro reaction in carbon dioxide absorbents. The reaction is accelerated in the presence of increased temperature and Baralyme, very low fresh gas flow, high sevoflurane concentration, and used absorbent. The reaction releases five compounds, of which compound A, fluoromethyl-2,2-difluoro-1-(trifluoromethyl) vinyl ether, is the most common.76 Nephrotoxic concentrations of compound A are believed to be >100 ppm; in children 1 MAC sevoflurane reaches 16 ppm after 5.6 MAC-hours in a circle circuit with a 2 L/min fresh gas flow.76 To date, there have been no instances of compound A–induced nephrotoxicity in children.
Inhalational anesthetics may also be degraded in the presence of desiccated carbon dioxide absorbent yielding carbon monoxide. Desiccation occurs when a large fresh gas flows through a carbon dioxide absorber for an extended period (>48 hours) without a reservoir bag attached. When a potent inhalational anesthetic contacts the desiccated absorbent, carbon monoxide is produced. The rate of production of carbon monoxide follows the order: Desflurane ≥ enflurane > isoflurane >> halothane = sevoflurane.77 This problem can be avoided if the AWS is turned off or the fresh gas is discontinued after each day or if the reservoir bag remains attached. Recently, carbon monoxide (≤18 ppm) was detected in the anesthesia breathing circuit in children although the source was unclear.78
Intravenous
Intravenous drugs are distributed first to the VRG, just as inhalational anesthetics are, and then to the muscle, vessel-poor group, and fat groups. The primary anesthetic effect occurs when the anesthetic reaches an adequate brain concentration; it is then redistributed to other tissues and metabolized to terminate its action. The pharmacokinetics of intravenous anesthetics depend on the dose and rate of drug administered, the binding of the drug in blood, the cardiac output and the distribution of cardiac output, metabolism, and excretion pathways.
Propofol
Diisopropylphenol is the most commonly used intravenous induction agent in children. This highly lipophilic drug distributes rapidly to the VRG to affect its anesthetic action. The effect site equilibration half-life (t1/2keo) has been estimated at 0.8 or 1.2 minutes, depending on the model.79 Its action is terminated by redistribution as well as hepatic and extrahepatic metabolism. Volume of distribution and clearance (to a lesser extent) decrease progressively during early childhood.80 However, clearance increases throughout gestation and the neonatal period, reaching 90% of adult values by 3 months of age.81 To maintain the 3 μg/mL blood concentration for anesthesia, a 50% greater induction and infusion dosing schedule is required in young children.82 The net effect is a context-sensitive half-life that increases with time in children more rapidly than in adults.82
The ED50 for loss of the eyelash reflex in children varies with the child’s age: 3 ± 0.2 mg/kg in infants 1 to 6 months; 1.3 to 1.6 mg/kg in children 1 to 12 years; 2.4 ± 0.1 mg/kg in children 10 to 16 years.83 The ED50 and ED90 of propofol to insert an LMA in children is 3.5 and 5.4 mg/kg (4.7 to 6.8 mg/kg 95% CI), respectively.84,85 The dose of propofol to facilitate tracheal intubation in children during sevoflurane anesthesia is 1 to 2 mg/kg.86,87
Propofol is an integral part of total intravenous anesthesia (TIVA) for maintenance of anesthesia in children undergoing medical/radiologic evaluations and surgery. For painless medical or radiologic (e.g., MRI) procedures in young children 2 to 6 years of age, an initial infusion rate of 15 mg/kg/hr (250 μg/kg/min) of propofol is recommended after either an inhalational induction or an IV induction.82,88,89 This dose may have to be increased to stop spontaneous movement, particularly if the children have neurocognitive impairment or are younger.90 Conversely, the infusion rate may be reduced in older children.
Based on pharmacokinetic modeling to maintain a blood concentration of 3 μg/mL, the infusion rate may be decreased during prolonged surgery to facilitate rapid emergence. The recommended stepwise reduction in the infusion rate in children 3 to 11 years of age after an intravenous induction with 2.5 mg/kg propofol is 15 mg/kg/hr (250 μg/kg/min) for 15 minutes followed by 13 mg/kg/hr (215 μg/kg/min) for 15 minutes, followed by 11 mg/kg/hr (180 μg/kg/min) for 30 minutes, followed by 10 mg/kg/hr (166 μg/kg/min) for 60 minutes, followed by 9 mg/kg/hr (150 μg/kg/min) for the next 2 hours.82 Target-controlled infusions (TCI) in children are available in Europe, but not in North America.91,92 These devices use preset algorithms based on the pharmacokinetics that use similar dosing algorithms with modest success.
Propofol causes pain in 70% or more of patients during intravenous induction of anesthesia93; the pain is greater when it is injected into a small vein (as in the hand) than in the arm.94 This pain is most reliably prevented by administering 70% nitrous oxide before propofol or by applying a mini-Bier block with 0.5 to 1 mg/kg IV lidocaine for 60 seconds.93,94,95
Propofol has profound effects on the airway. After a rapid induction dose, a transient apnea is followed by a return of spontaneous respiration. Propofol reduces the hypopharyngeal dimensions although patency of the upper airway is preserved.96 A jaw thrust maneuver re-establishes a patent airway, should obstruction occur.97 An important and unique property of propofol is the ease by which an LMA can be inserted. Propofol relaxes the upper pharyngeal muscles to facilitate acceptance of the LMA. Although propofol induces apnea, atelectasis during spontaneous respiration occurs less frequently than with tracheal intubation.98
Propofol is the only anesthetic that has antinausea properties that may be exploited for use in children with histories of nausea and vomiting or who are undergoing emetogenic surgery.99
Although propofol is used for both sedation and general anesthesia, long-term sedation in infants and children is not recommended with propofol, after reports of unexpected death during propofol sedation with >4 mg/kg/hr for >48 hours.100 In the United States, 21 children and 68 adults died in association with propofol administration over a 10-year period101 Whether these deaths were the result of the long-chain triglycerides or propofol or both has not been clarified. At least three reports of incipient propofol infusion syndrome (PRIS) have been reported in children after only a few hours of anesthesia.102,103,104 The smallest infusion rate reported to trigger PRIS was 1.9 to 2.6 mg/kg/hr.100 Currently, long-term sedation with propofol is avoided in infants and children, especially in those with suspected inflammatory responses including sepsis.
The package insert suggests that caution should be exercised when propofol is administered to children with egg and soy allergies. See section on Allergies for a full discussion.
Ketamine
Ketamine is a phencyclidine derivative that offers enormous flexibility in the clinical care of children. This anesthetic can be used as a premedication (orally, nasally, rectally, or intramuscularly), a general anesthetic induction agent (intravenously or intramuscularly), and maintenance agent as an infusion, as a sedative (intravenously or intramuscularly), or as a neuroaxial analgesic (caudal/epidural).
Ketamine is available as a racemic mixture, in which the S enantiomer is four times more potent than the R enantiomer. Ketamine is extremely lipophilic with a rapid onset of action, within 30 seconds, and maximum effect by 1 minute; the half-time to equilibrate in the effect site (brain) is 11 seconds.105 Effective blood concentration of ketamine for anesthesia is 3 μg/mL.106 Clearance of ketamine is reduced in neonates, but reaches adult levels by 6 months of age.107 Context-sensitive half-life for ketamine in a 10-kg child increases from 30 minutes after 1 hour to 55 minutes after 5 hours. Emergence after a prolonged infusion of ketamine, especially when combined with opioids and benzodiazepines, may be delayed. Ketamine is primarily metabolized via CYP450 3A4 to norketamine.
Oral ketamine may be used for premedication in a dose of 5 to 6 mg/kg.108 It may cause nausea and vomiting postoperatively; nightmares are not common by this route. Ketamine may also be given intranasally, although the porous nature of the cribriform plate raises concern regarding the potential neurotoxicity of ketamine if it reaches the brain directly via this route. The dose of intranasal (IN) ketamine (racemic mixture) is 3 to 6 mg/kg and for S-ketamine 2 mg/kg.109 The rectal ketamine dose for premedication is 5 to 10 mg/kg, with recovery increasing substantially in duration with larger doses of ketamine. For intramuscular use, 2 to 5 mg/kg ketamine sedates an uncooperative child in 3 to 5 minutes with a duration of action of 30 to 40 minutes.
General anesthesia may be induced with 1 to 2 mg/kg intravenously, a technique that is useful in children with cyanotic heart disease, septic shock, and conditions in which spontaneous respiration should be preserved (as in a child with an anterior mediastinal mass [AMM]). Ketamine may also be given as a continuous infusion after a single bolus loading dose of 2 mg/kg IV.91 The regimen consists of 11 mg/kg/hr for 20 minutes followed by 7 and 5 mg/kg/hr for the same periods, then 4 mg/kg/hr for the next hour, and 3.5 mg/kg/hr thereafter. If midazolam and nitrous oxide were added, the same regimen without 20 minutes at 11 mg/kg/hr would provide adequate sedation.91
Ketamine is used occasionally for perioperative analgesia and has made a small resurgence for this indication in children with obstructive sleep apnea (OSA).110 It is also used for neuroaxial analgesia. S-ketamine is the more potent and preferred enantiomer to administer. If ketamine is administered in a neuroaxial block, a preservative-free formulation should be used. Caution: The neurotoxic risk of the racemic mixture of ketamine in the epidural space has not been established.111
Side effects associated with ketamine include increased secretions, nystagmus, and nausea and vomiting. The last effect may occur in up to 33% of children. Nightmares and hallucinations have been reported after ketamine but appear to be very infrequent. Coadministration of midazolam and awakening in a dark, quiet environment may reduce the risk of nightmares postoperatively.112
Ketamine may be contraindicated in children with increased intracranial pressure and in those at risk for seizures (although it has been administered to many children in whom their seizures were under control).
Etomidate
This hypnotic anesthetic is infrequently used in children as its pharmacology has not been understood until recently. It is reserved for those who are hemodynamically unstable (e.g., septic shock). The dose of etomidate is 0.2 to 0.3 mg/kg intravenously in adults, with children requiring 0.3 mg/kg. The pharmacokinetics have only recently been estimated: With greater clearance and volume of distribution in young children, larger doses are required in this age group than older children.113 Although the elimination half-life or context-sensitive half-life of etomidate has not been reported in children, repeated doses or infusions have delayed emergence which limits the use of this medication. Because etomidate causes pain at the site of injection, pretreatment with IV lidocaine and mini-Bier block is advised.
The major impediment to the use of etomidate and to its approval in many countries has been the suppression of adrenal glands, particularly in critically ill patients.114
Recent molecular engineering has yielded solutions to both the adrenal suppression and prolonged emergence after infusions of etomidate. Although there are no studies in humans, carbomethoxyetomidate does not substantively suppress the adrenal gland and is rapidly metabolized by tissue esterases, with an elimination half-life not unlike remifentanil.115 If this new molecule proves safe to use in humans, this new compound may be extremely useful as an induction agent as well as a maintenance agent without suppressing the adrenal gland.
Neuromuscular Blocking Agents
With standard twitch devices readily available, every child who receives a muscle relaxant should be assessed for their twitch response before attempting to antagonize the neuromuscular
blockade. The role of neuromuscular agents in children has diminished in the past decade or more with the demise in routine use of succinylcholine and the adoption of propofol as the adjunctive medication to facilitate tracheal intubation after induction of anesthesia with sevoflurane.
blockade. The role of neuromuscular agents in children has diminished in the past decade or more with the demise in routine use of succinylcholine and the adoption of propofol as the adjunctive medication to facilitate tracheal intubation after induction of anesthesia with sevoflurane.
In Europe, the most common cause of anaphylaxis during anesthesia is muscle relaxants, with succinylcholine and rocuronium being the most common causes,116 although in children, latex was the most common cause (42%) followed by muscle relaxants (32%) and antibiotics (9%).116 The explanation for the frequency of anaphylaxis to relaxants in Europe remained elusive, although regional differences in the use of pholcodine, an over-the-counter cough medicine, suggested that epitopes in pholcodine (and certain cosmetics) were structurally similar to those in the aminosteroidal relaxants, which led to a cross-sensitivity and anaphylactic reactions, even upon first exposure.117 Indeed, after pholcodine was banned in Norway, the incidence of anaphylactic reactions to muscle relaxants in that country decreased dramatically suggesting that this over-the-counter cough medicine sensitized the population to the relaxants.
Succinylcholine
As the only depolarizing muscle relaxant in clinical practice, succinylcholine remains the agent that provides the most rapid onset and offset of paralysis, without additional medications to recover the normal twitch response. Succinylcholine comprises two acetylcholine molecules fused together; it acts by depolarizing the acetylcholine receptors of the neuromuscular endplate.
The intravenous dose of succinylcholine is 3 to 4 mg/kg in neonates and infants, 2 mg/kg in children, and 1 mg/kg in adolescents.118 The larger dose requirement with decreasing age has been attributed to the larger volume of distribution in younger infants. Paralysis usually occurs within 30 to 60 seconds and lasts approximately 5 minutes.118 In contrast, an intramuscular dose of 4 mg/kg paralyzes 100% of children within 1 to 2 minutes, although the duration may be as great as 20 minutes.119 Rarely is it necessary to administer succinylcholine intra- or sublingually, but this approach may be optimized with digital massage of the injection site.120 The speed of onset of paralysis when 1.1 mg/kg is administered intralingually is intermediate between IV and IM rates, ∼75 seconds.121 It is imperative to avoid midline sublingual blood vessels to avoid a sublingual hematoma. To administer succinylcholine either IM or sublingually, a small gauge (25G) needle should be used to minimize vascular trauma. This author routinely administers atropine 20 μg/kg before succinylcholine given via any route to prevent bradycardia and asystole after a single dose in infants and children.122
The action of succinylcholine is terminated by pseudocholinesterase (or plasma cholinesterase) which is located on 3q26.1 and 3q26.2.123 The residual products of metabolism have no neuromuscular activity. Pseudocholinesterase activity may be modified by a number of factors, inherited or acquired (Table 42-5). The inheritance pattern for pseudocholinesterase is autosomal recessive, which yields a host of phenotypes. Four alleles code for most of the genetic variants of pseudocholinesterase: “Usual” (U); “Atypical” (A); “Fluoride resistant” (F); and “Silent gene” (S).123 Several minor variants (H, J, and K) have also been reported. A second gene locus that codes for pseudocholinesterase in only 10% of Caucasians has been identified. It produces a C5 band on electrophoresis that yields 30% more pseudocholinesterase enzyme than normal (Neitlich variant).124 In addition, another gene variant with increased activity named E Cynthiana has been identified.125
Table 42-5. Pseudocholinesterase Variants | ||||||||||||||||||||||||
---|---|---|---|---|---|---|---|---|---|---|---|---|---|---|---|---|---|---|---|---|---|---|---|---|
|
The genetics of pseudocholinesterase activity variant follows simple Mendelian inheritance. Individuals may be homozygous or heterozygous for the gene. The vast majority of the population has normal responses to succinylcholine; that is, homozygous usual pseudocholinesterase, U/U. About 1:30 patients are heterozygous atypical (U/A) with minimal clinical prolongation of the duration of action of succinylcholine (∼15 minutes). In contrast, 1:3,000 to 1:10,000 patients are homozygous atypical (A/A) with a clinical phenotype manifesting a duration of action of 1 hour. In the case of fluoride-resistant, the frequency of homozygous F/F is 1:150,000, with a modest prolongation of succinylcholine activity of 1 to 2 hours. For the homozygous silent gene variant, S/S, the frequency is 1:10,000 patients, with a clinical duration of 6 to 8 hours. The other variants H, J, and K are associated with a 90%, 66%, and 30% reduction in pseudocholinesterase activity, respectively. The homozygous H variant yields the greatest duration of action of succinylcholine among these three 1 to 2 hours. The K variant is thought to occur in 13% of the population and the homozygous K variant occurs in 1:63, extending the duration of succinylcholine <1 hour. Interestingly, the K variant was present in 89% of A variants suggesting that more than one mutation is often present, for example, U/AK. The E Cynthiana and C5 variants destroy succinylcholine at an ultrarapid speed that may provide such a transient paralysis that the child recovers
before laryngoscopy is attempted. This variant was not widely considered during the era of succinylcholine-induced masseter muscle spasm. The management of prolonged response to succinylcholine is conservative. Sedation/anesthesia and ventilation should be maintained and blood sent for identification of the gene defect.
before laryngoscopy is attempted. This variant was not widely considered during the era of succinylcholine-induced masseter muscle spasm. The management of prolonged response to succinylcholine is conservative. Sedation/anesthesia and ventilation should be maintained and blood sent for identification of the gene defect.
Identification of the specific gene defect depends on the laboratory analysis of pseudocholinesterase activity and gene identification. The former involves incubating a sample of blood with dibucaine (an amide local anesthetic) that inhibits normal, but not abnormal pseudocholinesterase. When benzoylcholine is added to the blood, dibucaine suppresses the degradation of benzoylcholine by normal pseudocholinesterase by >71% (hence a dibucaine number of 71) whereas the degradation by A/A is only minimally depressed; that is, by 20%, hence the dibucaine number is 20. Intermediate inhibition is a dibucaine number of 60. A similar response occurs when fluoride is added to blood with normal pseudocholinesterase inhibited, but atypical appears resistant to it. Hence, a small dibucaine number suggests an abnormal pseudocholinesterase activity. Homozygote silent gene, S/S, conveyed no pseudocholinesterase activity, although variants of this defect also exist.123
Side Effects
The salient side effects associated with succinylcholine include arrhythmias (most notably bradycardia), rhabdomyolysis (with hyperkalemia and myoglobinuria), raised intraocular pressure, fasciculations, and malignant hyperthermia (MH).
Succinylcholine causes arrhythmias by acetylcholine-associated activation of the vagal nerves. Sinus bradycardia is the most common arrhythmia, which may progress to transient asystole after a single intravenous dose of succinylcholine in a child.126 Bradycardia can be prevented by administering an anticholinergic such as atropine (10 to 20 μg/kg) or glycopyrrolate (5 to 10 μg/kg) before succinylcholine.122
Rhabdomyolysis may occur when succinylcholine is administered to children with MH or a myopathy.127,128 The muscle breakdown releases massive concentrations of hyperkalemia as well as myoglobin, both resulting in potentially fatal consequences.
Hyperkalemia may occur in response to IV succinylcholine in children with a variety of disorders: Myopathies, upper and lower motor neuron disorders, burns, severe sepsis, and chronic immobilization (e.g., drug-induced, trauma-induced) (usually for weeks).127 There is no evidence that succinylcholine presents additional risks when administered to patients with renal failure.129 Treatment for hyperkalemia after succinylcholine is intravenous calcium (calcium chloride 10 mg/kg) that should be repeated until the arrhythmias (ventricular wide complex) dissipate and the rhythm returns to normal sinus rhythm.
Succinylcholine increases intraocular pressure 7 to 10 mm Hg reaching a peak pressure 1 to 2 minutes after IV administration and returns to the baseline in 5 to 7 minutes.130 This increase may be attenuated by pretreatment with anesthetics, although none provides 100% guarantee that the increase will not occur. In the presence of a lacerated globe, this increase in intraocular pressure may increase the extrusion of intraocular contents although greater increases in pressure may occur during crying and coughing.130
Fasciculations occur immediately after administration of IV succinylcholine. They have not been associated with any clinical sequelae. Some assert that fasciculations increase the risk of regurgitation by increasing the abdominal muscle tone. However, the crura of the diaphragm comprise skeletal muscle, which also fasciculates preventing any decrease in gastric barrier pressure. Adolescents with muscular builds are at an increased risk of developing postoperative muscle pains after succinylcholine. To prevent these pains, which can be serious, consideration should be given to avoid succinylcholine in this age group.
MH is a pharmacogenetic disorder of calcium metabolism in skeletal muscle.128 The triggers (succinylcholine and/or inhalational anesthetics) induce an exaggerated release of intracellular calcium, which causes sustained muscle contractions. These sustained contractions generate heat and muscle breakdown with the release of intracellular potassium, myoglobin, and CPK. The earliest sign of an MH reaction is an increase in end-tidal pCO2 that is accompanied by an increase in respiratory rate and hemoglobin desaturation. Late signs include increases in body temperature, disseminated intravascular coagulopathy, and sepsis. The definitive treatment for MH is intravenous dantrolene 2.5 mg/kg, repeated as needed until the reaction subsides.131 (See below and Chapter 23 for further details on MH.)
Rocuronium
Rocuronium is a steroidal muscle relaxant that is an analogue of vecuronium but differs from the latter by a more rapid onset of action and reduced potency. It is eliminated almost exclusively by the liver; hence liver failure may prolong its duration of action of rocuronium.132 In contrast, renal failure should have minimal effect on its elimination. Sevoflurane potentiates the action of rocuronium.
The potency of rocuronium is greatest in infants, least in children, and intermediate in adults. The dose should be adjusted according to the child’s age: The ED95 in infants is 0.25 mg/kg and in children, 0.4 mg/kg.133,134 In healthy children during sevoflurane anesthesia, 0.3 to 0.4 mg/kg rocuronium provides suitable intubating conditions in 2 to 3 minutes and permits antagonism within 20 minutes. Twice the ED95 or 0.6 mg/kg IV rocuronium provides relaxation in 1 to 1.5 minutes. Sevoflurane potentiates the effect of rocuronium compared with balanced anesthesia, a pharmacodynamic, not pharmacokinetic effect.135 The time to 90% recovery of the twitch response after 0.6 mg/kg IV is 46 minutes in children. Recovery after rocuronium in infants is prolonged compared with that in children as a result of the reduced clearance and increased volume of distribution in the former.134 At 3 to 4 × ED95, 0.9 to 1.2 mg/kg IV rocuronium yields similar intubating conditions to succinylcholine within 60 seconds and may be used for rapid sequence induction (RSI), although recovery may be markedly prolonged.136,137,138
Intramuscular rocuronium (1.8 mg/kg) has been recommended for emergency situations when an IV is not available. However, this dose and route provides poor intubating conditions after 4 minutes and a duration of action of 80 minutes. The author does not recommend this route and dose of administration.
Atracurium
Atracurium is a benzylisoquinolinium muscle relaxant that undergoes spontaneous degradation in blood primarily by Hofmann elimination yielding the major metabolite, laudanosine, which is devoid of neuromuscular blocking properties.132 It comprises 10 isomers. A typical intubating dose in infants and children is 0.5 mg/kg IV (2 to 3 × ED95) with an onset of 2 minutes and a duration of action of 15 to 30 minutes. Complete recovery is usually achieved within 45 to 60 minutes. With its brief half-life, atracurium is suited for use as a continuous infusion in a dose of 6 μg/kg/min during isoflurane anesthesia and 9 μg/kg/min during a balanced intravenous anesthetic.139 Renal and hepatic failures
do not affect the duration of action of atracurium. Side effects associated with atracurium include cutaneous erythema, bronchospasm, and wheezing after a rapid large bolus administration; rarely has anaphylaxis been reported.
do not affect the duration of action of atracurium. Side effects associated with atracurium include cutaneous erythema, bronchospasm, and wheezing after a rapid large bolus administration; rarely has anaphylaxis been reported.
Cis-atracurium
Cis-atracurium is one of the 10 isomers of atracurium that has supplanted the use of atracurium. It has a potency that is threefold greater than atracurium resulting in more specificity for the receptor and fewer side effects such as histamine release.132 It too is degraded by Hofmann elimination with a typical duration of action of 30 to 50 minutes. Suitable intubating conditions are achieved with 150 μg/kg (3 × ED95) by 2 minutes after the dose.140 Renal and hepatic failures do not affect the duration of action of cis-atracurium. Side effects associated with the administration of cis-atracurium are minimal.
Neostigmine
This author strongly recommends antagonizing all neuromuscular blocking agents in infants and children when extubation is planned,141 provided the time interval from the last dose has not exceeded 2 hours (in most cases). The train-of-four should be ≥0.9 before the trachea is extubated.141 Any child who appears weak, as a “fish out of water,” requires antagonism or a repeat dose of antagonism of the neuromuscular blockade. In order to successfully antagonize the relaxant, vital signs including temperature must be normal.
Neostigmine is an anticholinesterase compound that antagonizes neuromuscular blockade by preventing the degradation of acetylcholine. The acetylcholine competitively displaces the muscle relaxant from the neuromuscular junction. The dose of neostigmine in infants and children is 30% to 40% less than that in adults, or 20 to 40 μg/kg, which should be administered when at least one twitch is present in the train-of-four. If the recovery of neuromuscular blockade is incomplete, repeat doses of neostigmine may be administered up to 70 μg/kg. Care must be taken to avoid exceeding 100 μg/kg as acetylcholine-associated weakness may occur.
Neostigmine should be preceded by an anticholinergic, atropine 20 μg/kg or glycopyrrolate 10 μg/kg, to minimize the effect of neostigmine on the nicotinic receptors. Atropine causes a greater increase in heart rate but has a shorter duration of action than glycopyrrolate.
Sugammadex
This γ-cyclodextrin compound is a cylindrical oligosaccharide that uniquely binds rocuronium (and to a lesser extent vecuronium) to eliminate its activity.142 When administered in the presence of moderate to profound rocuronium-induced neuromuscular blockade, sugammadex restored the twitch response thereby providing a direct intervention for a “cannot ventilate, cannot intubate” situation. The rocuronium/sugammadex complex is excreted unchanged in the kidney. Sugammadex has been used extensively in Europe but is not approved in North America due to several episodes of hypersensitivity in preclinical trials. In children and adolescents, a single dose of ≥2 mg/kg sugammadex after partial recovery (two twitches of the train-of-four) from neuromuscular blockade with rocuronium yielded a train-of-four of 0.9 in approximately 2 minutes.143 Dose response studies in children are required. Most recently, two reports of sugammadex reversal of rocuronium-induced anaphylaxis refractory to vasopressors suggest another important clinical role for sugammadex.144
Opioids
Morphine
Opioid use in infants and children has increased dramatically in the past few decades, in response to recognition that children experience pain and that pain adversely affects the speed and quality of recovery.
In children, perioperative analgesia is accomplished with intraoperative IV doses of 50 to 100 μg/kg and postoperative doses of 50 μg/kg morphine.145 Perioperative infusions of morphine may also be administered by diluting morphine (1 mg/kg of the child’s weight) in 100 mL lactated Ringer (LR) solution and infusing at 1 to 4 mL/hr. This provides analgesic blood concentrations of morphine, 10 to 40 ng/mL.145 Morphine may also be administered via the caudal/epidural route. Doses of 25 to 50 μg/kg provide prolonged analgesia with a small incidence of side effects (vomiting, pruritus).145 Oral morphine has also been administered, although its bioavailability is only 35% due to the first-pass effect.
Side effects after morphine include dose-dependent respiratory depression and incidence of vomiting (particularly at >100 μg/kg). Histamine release and urticaria at the site of injection are local, nonimmunologic reactions.
Fentanyl
This semi-synthetic opioid is the most widely used intraoperative analgesic in children. This very lipid soluble opioid, which is bound primarily to α1-acid glycoprotein in blood, has a very rapid onset of action, hemodynamic stability, and brief duration of action after a single dose.146 It may be used via the IV, IM, oral, IN, and caudal/epidural routes. It is 50 to 100 times more potent than morphine, with doses 1 to 3 μg/kg IV attenuating the sympathetic responses to minor surgical procedures, up to doses of 12 to 50 μg/kg for neonatal surgery.147 Fentanyl is infrequently administered IM; oral fentanyl, 10 to 20 μg/kg, is used in breakthrough cancer pain. IN fentanyl (1 to 2 μg/kg) has been used as a premedication and to reduce agitation in children undergoing myringotomy and tube surgery.109,146 Epidural fentanyl has been administered to children via the epidural space in a dose of 1 to 2 μg/mL to supplement local anesthetics. Although there is very little evidence that fentanyl augments the analgesia provided by a lumbar epidural block in a child with an effective local anesthetic concentration (e.g., 0.125% bupivacaine or 0.0625% levobupivacaine),148 it is commonly added resulting in pruritus, nausea/vomiting, and urinary retention.
The action of clinical doses of fentanyl is terminated by redistribution and, secondarily, by clearance in the liver.146 The initial redistribution is rapid; however, once tissue binding sites become saturated, the elimination half-life of fentanyl increases.149 metabolized extensively by CYP450 3A4 to inactive metabolites. The context-sensitive half-life of fentanyl in adults after a brief infusion for 1 hour, 20 minutes, increases dramatically to 4 hours after an 8-hour infusion.149 Elimination half-life may exceed 20 hours after a chronic infusion. To offset this increasing context-sensitive half-life, the dose of fentanyl must be adjusted. After a prolonged infusion of fentanyl, it is necessary to slowly taper the dose and monitor for opioid withdrawal.
Meperidine
Meperidine is no longer recommended as an analgesic because of the risk of seizures (from normeperidine) and the accumulation of normeperidine after repeated doses of meperidine. It is currently recommended only for shivering.146 The dose of meperidine for analgesia is 1 to 2 mg/kg and for shivering 25% to 50% of that dose. The elimination half-life of meperidine in children is 3 hours.146
Remifentanil
Remifentanil is a unique μ-receptor opioid that undergoes spontaneous degradation in blood by tissue esterases, with an elimination half-life of approximately 5 minutes that is independent of the duration of infusion.150 With this brief half-life, remifentanil is usually administered as a continuous infusion. The context-sensitive half-life (the time to decrease the blood concentration by 50%) of remifentanil is 3 to 8 minutes. Metabolites of remifentanil are effectively nontherapeutic.
Remifentanil is 10- to 60-fold more potent than alfentanil.150,151 The infusion rate for remifentanil ranges from 0.05 to 0.25 μg/kg/min (although larger doses have been administered), with the dose adjusted according to the presence of concomitant medications (e.g., inhalational anesthetics). A loading dose (0.1 to 0.2 μg/kg) is usually not required as the infusion rapidly establishes an effective target organ concentration and hypotension is a real possibility.
When administered in large doses, remifentanil may cause hypotension, bradycardia, and chest wall rigidity, although the latter may be more likely vocal cord closure rather than chest rigidity.151 Prolonged administration has resulted in tachyphylaxis. Recent evidence suggests that nitrous oxide may attenuate the risk of tachyphylaxis.152
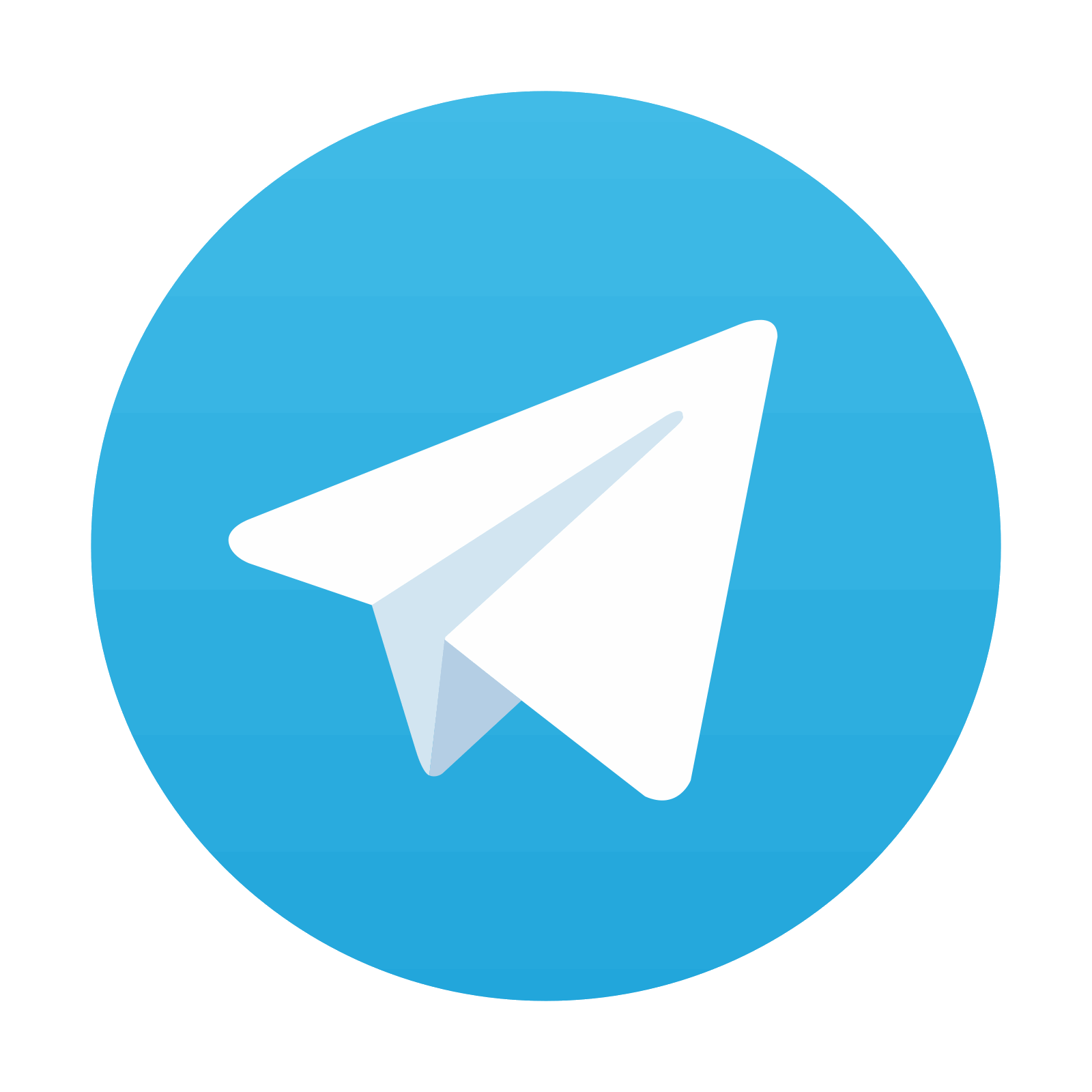
Stay updated, free articles. Join our Telegram channel
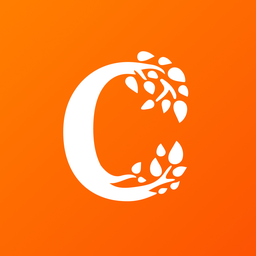
Full access? Get Clinical Tree
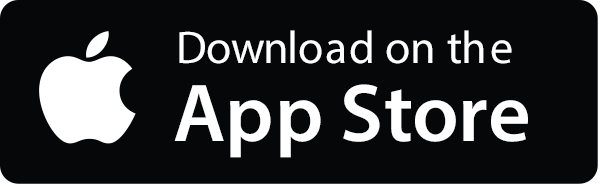
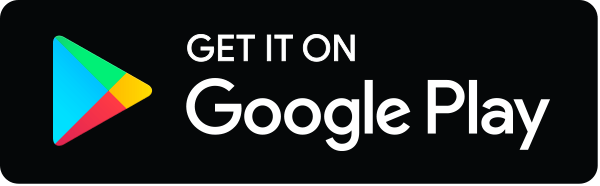
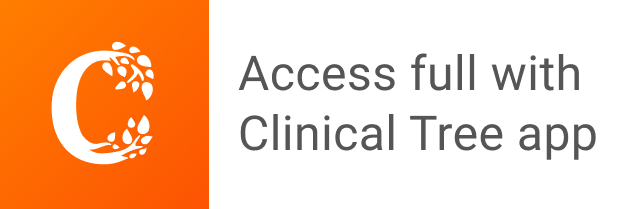