Pathophysiology of Cardiac Arrest
Karl B. Kern
The mechanism of blood-flow generation during cardiopulmonary resuscitation (CPR) is complex and depends on body habitus, the duration of CPR and the CPR technique utilized. Cardiac compression predominates as the mechanism early in CPR, with the thoracic pump mechanism more predominant as time progresses.
Current concepts of the physiology of blood flow during CPR and their clinical implications
Coronary perfusion pressure as a major determinant of resuscitation outcome
Mechanisms for optimizing coronary perfusion pressure during CPR
Cardiac dysfunction and the CPR stunned myocardium: implications for managementas part of the post–circulatory arrest syndrome
Introduction
Cardiac arrest causes the complete cessation of forward blood flow, with resultant global ischemia affecting the entire organism. Various tissues have different tolerances to such global ischemia, but all will eventually succumb if blood flow is not restored in a timely fashion. The most prominent injuries occur in the central nervous system, which initially responds to ischemia with loss of consciousness after only 7 to 10 seconds of an circulatory arrest. Likewise, once the myocardial cells become globally ischemic, cellular function, including contraction, stops; if blood flow is not restored, myocytes begin to autoinfarct. The duration of no blood flow is crucial in these responses; hence the importance of CPR for restoring at least some modicum of flow during cardiac arrest. CPR restores only a portion of normal blood flow previously generated by a contracting heart. Nonetheless, such support is crucial for the survival of both the central nervous system and the myocardium.
The Mechanism of Blood Flow During CPR
The mechanism of blood flow produced during CPR has been a controversial and debated topic for several decades. Just how blood flow is produced became an important issue in trying to determine the optimal external chest compression rate. In 1977, Taylor and colleagues suggested that within the range of 40 to 80 compressions per minute, the duration of chest compression was more important than the rate of compression.1 This ratio of the duration of compression to decompression is the “duty cycle.” A duty cycle of 50% (50% of the time the chest is compressed and 50% decompressed, generally by passive recoil) with 60 compressions per minute was initially thought optimal. In opposition to this concept, investigators at Duke University found that a higher chest compression rate produced better blood flow during closed chest CPR, up to a maximum rate of 140 compressions per minute.2 Above a compression rate of 140/min, the relaxation or diastolic period for coronary filling is compromised to the point where total myocardial blood flow diminishes. In recognition of these findings, the National Conference on CPR in 1985 recommended increasing the chest compressions to 80 to 100/min.3
Cardiac or Thoracic Pump Mechanism?
Physicians and scientists alike have assumed that external chest compression produces temporary blood flow by compressing the heart between the sternum and the vertebral column.
Physicians and scientists alike have assumed that external chest compression produces temporary blood flow by compressing the heart, similar to the manner of open chest internal cardiac massage, with the heart being squeezed between the sternum and the vertebral column. Indeed, a famous illustration by Frank Netter suggests such a mechanism, with the heart being squeezed between the sternum and the vertebral column and competent, functional valves directing the resultant blood flow out the aortic valve and to the body. It has been assumed that with such cardiac compression and competent mitral and aortic valves, blood moved in an antegrade fashion from the left ventricle into the aorta. This widely held concept was challenged in the late 1970s and early 1980s.4 Some investigators felt that the concept of cardiac compression and competent valvular function was inconsistent with the number of observations during resuscitation. They noted that when sternal compression was performed in a patient with a flail chest, no arterial blood pressure was recorded until the chest was bound to prevent paradoxical expansion.5 They also observed that patients with severe chronic obstructive pulmonary disease with marked increases in their anterior–posterior chest diameter and relatively small hearts could nonetheless be resuscitated by sternal compression. Finally, they observed that during conventional CPR, the compression cycle that followed ventilation often resulted in an increased blood pressure and carotid blood flow. To them, these observations appeared inconsistent with direct cardiac compression from external chest compression, and a new potential mechanism for blood-flow generation during CPR was developed. The “thoracic pump” mechanism of blood-flow generation suggested that forward blood flow occurring during CPR was due to an increase in intrathoracic pressure compared with extrathoracic pressure. This theory was also supported by the work of Criley et al. on “cough CPR.”6
Cough Cardiopulmonary Resuscitation
Cough CPR consists of having a patient with a recent onset of asystole or ventricular fibrillation forcefully cough every second.7 Since no hands are placed on the patient, this is an excellent example of the power of the thoracic pump mechanism to produce blood flow during CPR. If the patient coughs forcefully every second, significant aortic systolic pressures can occur. These pressures are often enough to perfuse the brain and consciousness can be preserved. The obvious disadvantage of this technique is that it must be initiated before unconsciousness occurs. Cough CPR has been most successfully employed in the cardiac catheterization suite, where Criley et al. have reported examples of patients sustaining consciousness for up to 40 seconds after the onset of ventricular fibrillation.6 Although some lay publications have touted this technique as a way to perform CPR on oneself, cough CPR has generally failed to meet its initial expectations outside the cardiac catheterization laboratory.8
The Thoracic Pump Mechanism
An important rediscovery was that blood pressures in the aorta and right atrium were often similar during external chest compressions. This observation was initially reported by Weal and Rockwell-Jackson in 1962 but at the time received little attention.9 Nearly two decades later, investigators at Johns Hopkins noted that during external chest compressions, central venous and aortic pressures were often similar; indeed, pressures in all cardiac chambers and the intrapleural space were nearly equal. If the cardiac compression mechanism is responsible for blood flow during CPR, the fluid-filled systems of the heart and great vessels must contain a pressure gradient across a resistance for the production of forward blood flow. Under the normal physiology occurring during sinus rhythm, there is a large pressure gradient involving the aorta, right atrium, and central venous systems. The lack of such a gradient during external chest compression suggests the heart is not functioning strictly as a pump but that the entire thorax was indeed the pump.10
In contrast to the intrathoracic structures with similar pressures, the investigators at Johns Hopkins found a
significant pressure difference between the extrathoracic carotid artery and the intrathoracic cardiac chambers and the right atrium and the extrathoracic jugular veins. This pressure gradient was thought to be responsible for producing the forward cerebral blood flow. It is at this time that Criley et al. demonstrated that the jugular venous valves were operative during cough CPR.11 Indeed, early anatomists had also appreciated the presence of internal jugular venous valves and had described them in the 1950s.12
significant pressure difference between the extrathoracic carotid artery and the intrathoracic cardiac chambers and the right atrium and the extrathoracic jugular veins. This pressure gradient was thought to be responsible for producing the forward cerebral blood flow. It is at this time that Criley et al. demonstrated that the jugular venous valves were operative during cough CPR.11 Indeed, early anatomists had also appreciated the presence of internal jugular venous valves and had described them in the 1950s.12
Further supports for the theory that increases in intrathoracic pressure create forward blood flow during external chest compression came in the 1980s from echocardiographic studies of clinical CPR.13,14 Two different two-dimensional echocardiographic studies performed late in a protracted resuscitation effort, showed that the mitral valve was not competent and did not close in a consistent fashion and that the left ventricular internal diameter was not deformed with chest compressions.13,14 These findings suggest that increased intrathoracic pressure in some patients, not cardiac compression, accounted for forward blood flow during chest compressions.
Nonetheless, cardiac compression does occur in some humans.5 In a few patients studied at Johns Hopkins, central venous pressures were significantly lower than arterial pressure during external chest compression, indicating a pressure gradient and the possibility that in these patients a cardiac compression mechanism for blood flow during CPR was possible.
Based on this new theory for blood-flow generation during external chest compression, the investigators at Purdue University attempted to apply this concept to improving alternative techniques to conventional CPR. The group explored two basic strategies, simultaneous chest compression and ventilation, as well as abdominal binding.15 Increased intrathoracic pressure could be obtained by clamping the endotracheal tube during chest compressions; however, the initial increase in pressure and flow dissipated rapidly, secondary to the inhibition of venous return by high intrathoracic pressure. Maintaining inflated lungs during external chest compressions, these investigators found significant increases in arterial pressure and carotid flow over those observed with conventional CPR.10 Of particular interest, in their experimental model large animals (i.e., canines), treatment with simultaneous high-pressure ventilation and chest compressions produced significant systolic flows in the carotid arteries. However, the same was not true in smaller dogs.16 In the smaller dogs, cardiac compression evidently occurs with relatively good blood-flow generation, and the addition of simultaneous high-pressure ventilation did not appear to improve these hemodynamics. However, in large animals, in which cardiac compression plays a smaller role, if any, the addition of simultaneous ventilation clearly improved the peripheral circulation during CPR.10 Abdominal binding was shown by Redding et al. to improve hemodynamics during CPR.17 Unfortunately, further investigations of this technique reported increases in liver lacerations secondary to the abdominal binding, and the technique fell out of favor. It was also noted that abdominal binding had a detrimental effect on coronary perfusion pressure by the consistent increase in right atrial pressure both in systole and diastole.
The ultimate test for the presence and efficacy of the “thoracic pump mechanism” as employed using simultaneous chest compressions and high-pressure ventilation with or without abdominal binding is its effect on survival. In an effort to evaluate this effect on survival, simultaneous chest compression and ventilation CPR with abdominal binding was compared with standard CPR in a laboratory experiment at the University of Arizona.18 Survival in the control group of canines receiving standard CPR was 5 out of 6, whereas none of the 6 animals undergoing simultaneous chest compression and high-pressure ventilation had return of spontaneous circulation in spite of intensive efforts.18 A clinical trial with simultaneous compression–ventilation CPR was also performed.19 This clinical study enrolled nearly a thousand patients in an out-of-hospital cardiac arrest trial where ambulance staff were randomly assigned to use simultaneous compression–ventilation CPR or conventional CPR. Both hospital admission and survival to discharge were greater in the conventional CPR group than in the experimental group receiving simultaneous compression–ventilation CPR (P ≤ 0.01). In the subset of adult patients with nontraumatic witnessed cardiac arrest, survival was 35% of 337 versus 23% of 365 (P ≤ 0.001). These two studies have dampened enthusiasm for simultaneous chest compression–ventilation CPR, and this technique is not currently recommended.
Other investigators were less convinced about the importance of the thoracic pump mechanism for blood-flow generation during resuscitation efforts. Rankin and colleagues at Duke University were convinced, from their own clinical experience, that rapid chest compression rates were more effective than slower rates and that this reflected a cardiac compression mechanism for blood-flow generation. These investigators studied the effects of varying manual chest compression rates, force, and durations in large chronically instrumented dogs.2,20 In their model, they found that the relative contribution of thoracic pump and direct cardiac compression mechanisms to blood flow varied depending on the method of CPR being performed. During high-impulse (increased frequency) CPR, direct cardiac compression seems to be the predominant mechanism, while during low-momentum compression techniques, the thoracic pump mechanism seemed to predominate. At the same time, new echocardiographic experimental studies by Weil et al. supported the cardiac/vascular compression theory of CPR-generated blood flow.21 In fact, echocardiographic studies in anesthetized minipigs demonstrated incompetent cardiac valve motion with a clear change in left ventricular dimensions during the early phases of cardiac arrest with closed-chest CPR. The investigators interpreted these data as further evidence for direct cardiac compression in the early phases of cardiac arrest and resuscitation efforts. Since that time, additional transesophageal echocardiographic studies in experimental models of cardiac arrest22 and clinical cases23 have also shown left ventricular compression in competent mitral valve function, again suggesting a cardiac compression mechanism for blood flow.
Current Concepts on Blood Flow During CPR
As in most disagreements, there appears to be truth on both sides of this controversy. Cardiac compression clearly occurs at times, while the thoracic pump mechanism for blood-flow generation during CPR also appears to be operational, particularly later in the resuscitation effort. Open-chest cardiac massage and high-impulse closed-chest compression in small subjects undoubtedly produce blood flow predominantly by cardiac or vascular compression. Whereas cough and “vest CPR” forms of CPR, both of which produce very little thoracic compression, but rather large fluctuations in intrathoracic pressure, appear to produce blood flow via the thoracic pump mechanism.
The authors examined this at the University of Arizona using our large database of experimental CPR studies. On subjects undergoing open-chest cardiac compression, the authors observed that there is a large difference between the aortic and right atrial systolic pressures. In contrast, the authors found little or no difference in systolic pressure between the aorta and right atrium in experimental subjects in whom blood is generated by the thoracic pump mechanism during vest CPR. This led to the conclusion that the absolute difference between aortic and right atrial systolic pressure, which we termed the systolic pressure gradient, could actually indicate the mechanism of blood flow in real time. The authors reviewed 63 experiments using a variety of CPR techniques and animal sizes. Each underwent 3 minutes of untreated ventricular fibrillation and then one of the five different types of CPR was initiated. Systolic pressure gradients between the aortic and right atrium were measured at 1, 7, and 17 minutes of the resuscitation effort.24 The systolic pressure gradient was greatest during open-chest cardiac massage (true cardiac compression), intermediate with external mechanical or manual standard CPR, and lowest with CPR performed with a vest apparatus (predominantly thoracic pump). With open-chest cardiac massage, this gradient was 60 to 65 mm Hg; with standard CPR in small animals, it was 28 to 30 mm Hg; and in larger animals, it was 15 to 20 mm Hg. With vest CPR, the gradient was only 2 to 5 mm Hg. In this particular series, it was also noted that 24-hour survival was greatest in those animals where cardiac compression mechanisms seemed to be the predominant mechanism of blood-flow generation. This is of interest in light of a report from Deshmukh and associates using 2D echocardiography to evaluate eight minipigs during CPR.21 They found aortic and mitral valves demonstrating competency (not wide open with constant leakage) during the first 5 minutes of CPR in all animals. In the three successfully resuscitated animals, valve competency persisted for the full 12 minutes of CPR.
It appears that the mechanism of blood flow during CPR can vary accorded to the CPR technique utilized and the duration of cardiac arrest.
It appears that the mechanism of blood flow during cardiopulmonary resuscitation can vary accorded to the CPR technique utilized. Cardiac/vascular compression is greatest with open-chest cardiac massage or high-impulse CPR, particularly in smaller subjects, while it is lowest with cough CPR or vest CPR. The mechanism of blood-flow generation also seems to vary with the duration of CPR efforts. Cardiac compression predominates as the mechanism early in CPR, with the thoracic pump mechanism more predominant as time progresses. This is consistent with the reported clinical echocardiographic reports noted earlier.13,14,23 In summary, the mechanism of blood-flow generation during CPR depends on body habitus, the duration of CPR, and the CPR technique utilized. Both mechanisms appear feasible and are probably present in each subject resuscitated.
Importance of Coronary Perfusion Pressure
“The basic problem, then, in resuscitations seems to us to be that of securing some means of some infusion—a coronary pressure, approximately amounting to 30 to 40 mm Hg.”
Experimental work in resuscitation research in the 1970s and 1980s seemingly focused on the physiologic mechanisms for creating systemic blood flow during closed-chest resuscitation.25,26 During that era, the importance of blood flow to sustain the myocardium and central nervous system during CPR became evident. Using microsphere techniques to measure blood flow, investigators found that regional perfusion of vital organs occurs with closed-chest compression CPR, but at substantially lower levels than during normal sinus rhythm.27,28,29 Central nervous system flows average about 30% of normal with good anteroposterior chest compressions. Myocardial blood flows achieved with external chest compressions average even less, generally only 10% to 20% of normal. Peripheral perfusion is almost nonexistent during CPR. Nevertheless, good CPR efforts can temporarily provide at least some perfusion to the myocardium and cerebrum until more definitive treatment (i.e., defibrillation) can be accomplished.
Myocardial perfusion during cardiac arrest can be estimated by measuring “coronary perfusion pressure” during the resuscitation effort. This perfusion pressure gradient correlates well with resultant cerebral and myocardial blood flows generated with CPR, and with the subsequent possibility of successful defibrillation and resuscitation.27,28
Determinants of Coronary Perfusion Pressure During Cardiopulmonary Resuscitation
Adequate Perfusion Pressure
The importance of an adequate perfusion pressure for successful resuscitation was first described by Crile and Dolley in 1906.30 While studying means of reversing cardiorespiratory
arrest in dogs and cats asphyxiated with the anesthetics of that time (chloroform and ether), they noted that “the basic problem, then, in resuscitations seems to us to be that of securing some means of some infusion—a coronary pressure, approximately amounting to 30 to 40 mm Hg.” This concept was further developed through the work of Redding and Pearson.17,31,32,33 These investigators showed that when aortic diastolic pressure during resuscitation was ≥40 mm Hg, animals could be successfully revived from cardiac arrest; but this was not possible if that level of diastolic pressure was not achieved. From their early work they deduced that the mechanism of action for epinephrine and other alpha-adrenergic agonists during cardiac arrest was to cause peripheral vasoconstriction, which raised the central aortic diastolic pressure and thereby increased coronary perfusion. Otto and coworkers confirmed this mechanism. By using selective blockade, these investigators evaluated the role of the alpha- and beta-adrenergic receptors during resuscitation. Animals that were blocked with the former had difficulty in responding to epinephrine (i.e., diastolic aortic pressure did not increase and they were not resuscitated). In contrast, those that were blocked with the latter were successfully resuscitated and exhibited peripheral vasoconstriction with elevated aortic diastolic pressures in response to adrenergic agonists.34,35,36
arrest in dogs and cats asphyxiated with the anesthetics of that time (chloroform and ether), they noted that “the basic problem, then, in resuscitations seems to us to be that of securing some means of some infusion—a coronary pressure, approximately amounting to 30 to 40 mm Hg.” This concept was further developed through the work of Redding and Pearson.17,31,32,33 These investigators showed that when aortic diastolic pressure during resuscitation was ≥40 mm Hg, animals could be successfully revived from cardiac arrest; but this was not possible if that level of diastolic pressure was not achieved. From their early work they deduced that the mechanism of action for epinephrine and other alpha-adrenergic agonists during cardiac arrest was to cause peripheral vasoconstriction, which raised the central aortic diastolic pressure and thereby increased coronary perfusion. Otto and coworkers confirmed this mechanism. By using selective blockade, these investigators evaluated the role of the alpha- and beta-adrenergic receptors during resuscitation. Animals that were blocked with the former had difficulty in responding to epinephrine (i.e., diastolic aortic pressure did not increase and they were not resuscitated). In contrast, those that were blocked with the latter were successfully resuscitated and exhibited peripheral vasoconstriction with elevated aortic diastolic pressures in response to adrenergic agonists.34,35,36
Perfusion Pressure Gradient
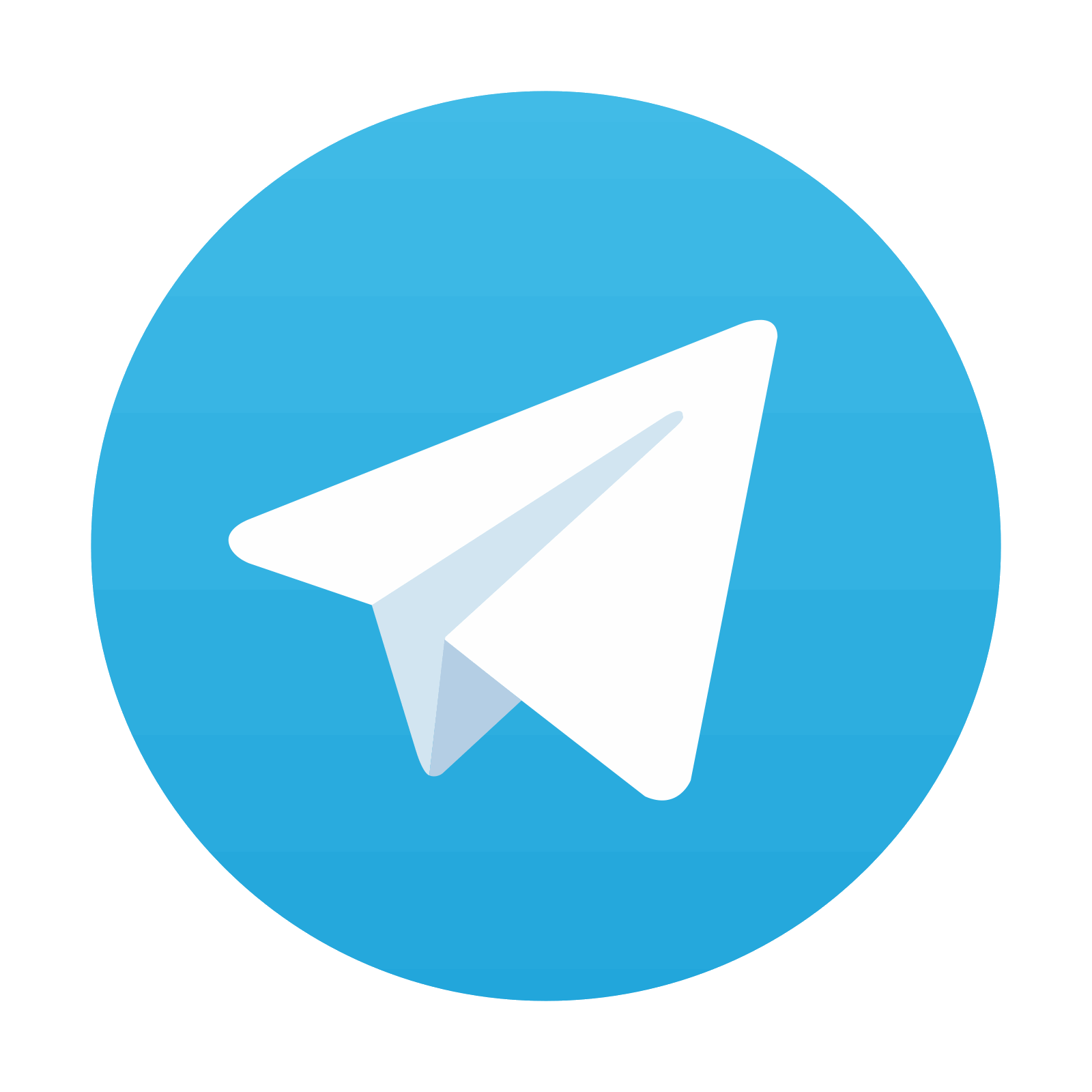
Stay updated, free articles. Join our Telegram channel
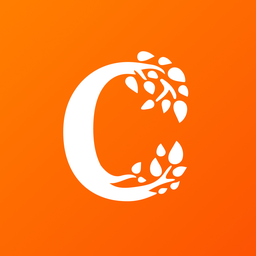
Full access? Get Clinical Tree
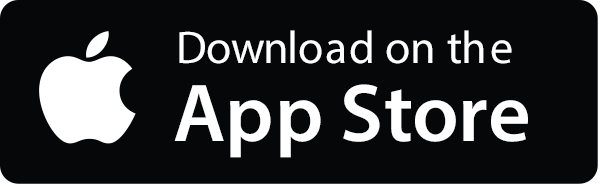
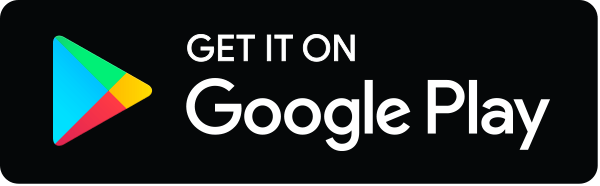
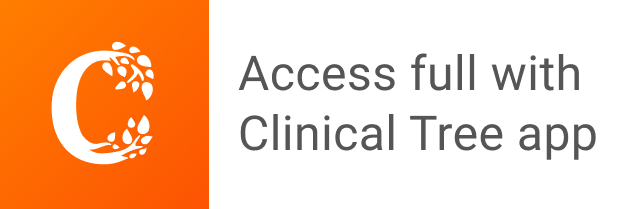