Fig. 7.1
A schematic of a model of O2 delivery and consumption during VV-ECMO. Part of the blood returning from the body with a low oxygen content (Cv) is diverted to the membrane lung (ML). In the ML lung the blood is fully saturated (Cout) and returned to the rest of the venous return. Cin and Cout are the oxygen content of the blood entering and exiting the ML, respectively. In the presence of recirculation, Cin is higher than Cv. The blood entering the lung (mixed venous blood (Cmv)) is determined by ECMO blood flow (ECBF) relative to patients’ cardiac output (CO). The arterial blood (Ca) is determined by the intrapulmonary shunt (NL shunt) relative to CO. An example is reported with relevant gas analytic values and oxygen content computation. The total oxygen delivery corresponds to total patient’s oxygen consumption (VO2) and is the sum of the oxygen contribution of the NL (VO2NL) and of ML (VO2NL). A more detailed description is provided in the text
7.2.2.1 Oxygen Delivery During VV-ECMO
During VV-ECMO, the arterial oxygen content (CaO2) will depend on the O2 delivering contributions of both the ML and the NL (Fig. 7.1).
Venous blood returns from the peripheral tissues with low oxygen content (CvO2). The blood pump generates the extracorporeal blood flow (ECBF) diverting part of the venous return, i.e. cardiac output (CO), towards the ML. The ML loads the ECBF with oxygen, raising the oxygen content from the inlet (CinO2) to the outlet (CoutO2) of an amount corresponding to the oxygen delivery of the ML (VO2ML = ECBF * (CoutO2 − CinO2)).
The VO2ML and then the contribution of ML to total oxygen delivery depend on three main factors:
- 1.
The ECBF is the most important factor: VO2ML will increase directly with the increase in ECBF. However, depending on intrinsic characteristics of the membrane lung, there is a limit to the oxygenator flow (“rated flow”) above which no more O2 can be added to the blood.
- 2.
The O2 pressure gradient between the extracorporeal sweep gas flow (ECGF) and the inlet blood: on the gas side, the O2 partial pressure depends on the FiO2 of the ECGF. On the blood side, the higher the CinO2, the lower the quantity of O2 that can be added to the blood. If the drainage and the returning cannula are too close or in specific cannula configuration (e.g. drainage from the jugular vein and return in the femoral vein), part of the already oxygenated ECBF is sucked back into the ECMO circuit. This phenomenon is called recirculation. The main effect of recirculation is to increase CinO2 and decrease VO2ML and then the oxygenation efficiency of the system.
- 3.
The intrinsic oxygenation capability of the ML which depends on the membrane diffusion characteristics (thickness, material) and the membrane surface area.
- 4.
The O2-bonding capability of the blood: It is important to remember that the main determinants of blood O2 content are haemoglobin saturation and haemoglobin concentration. The higher the haemoglobin concentration, the higher the amount of O2 that can be bounded and then transferred from the ML to the blood.
The oxygenated ECBF is then returned to the venous blood and directed towards the right heart. The oxygen content of the blood returning to the lung (the mixed venous blood, CmvO2) is the flow-weighted average of CoutO2 and CvO2: CmvO2 = [CoutO2*ECBF+ CvO2*(CO − ECBF)]/CO. In practice, the resulting effect of the membrane lung is to increase the O2 content of the blood returning to the lung from CvO2 to CmvO2. With the given CvO2, CmvO2 and then the mixed O2 saturation are directly proportional to ratio between ECBF and CO.
The oxygen contribution of the native lung (VO2NL = (CaO2 − CmvO2)*CO) depends on its residual gas exchange capability which depends on both the severity of the lung disease (i.e. the intrapulmonary shunt fraction) and the ventilator management.
The sum of VO2NL and VO2ML, i.e. the total oxygen delivered, corresponds to the total oxygen consumption of the patient (VO2Tot = VO2NL + VO2ML). Consequently, the lower the NL contribution, the higher must be the contribution of the ML and then the oxygen delivery efficiency of the ECMO system. A highly efficient VV-ECMO system, able to deliver a high VO2ML, requires high ECBF, minimal recirculation and low CinO2.
7.2.2.2 Effect of VV-ECMO on CO 2 Removal
While the efficiency in delivering O2 has several limitations, removal of CO2 during VV-ECMO is much easier to achieve. While the amount of O2 delivery is limited by full saturation of arterialized blood and by haemoglobin concentration, a substantial amount of CO2 can be removed from venous blood. In fact, normal venous blood carries around 50 mL of CO2/100 mL of blood, most of which in the form of bicarbonate ion. When the ECBF flows through the ML, dissolved CO2 is transferred from the blood to the gas side, while new dissolved CO2 is released from carbonic ion assuring a continuous availability of dissolved transferable CO2. In this process, the main limiting factor to the amount of removable CO2 is the partial pressure of CO2 on the gas side, which depends on the ECGF. High ECGF will maintain the partial pressure of CO2 on the gas side close to zero, thus maintaining a high CO2 partial pressure gradient. Indeed, contrary to oxygen delivery, efficient CO2 removal can be obtained with relatively lower ECBF.
7.2.3 Cannulation and Cannula Configurations for VV-ECMO
Achieving proper vascular access is a fundamental step in implementing an ECMO treatment [9–15]. An important historical step in cannulation for ECMO has been the availability of thin-walled cannulas, in the early 1990s, that allowed to move from surgical to percutaneous cannulation techniques. The percutaneous approach has several advantages: reduced risk of bleeding, shorter operative time and easier mobilization and nursing of the patient. This reason has become the standard technique for VV-ECMO [9–15].
Choice of vessels, cannulas type and size and configuration are mainly dictated by the maximum ECBF needed for the support of the patient, the maximum recirculation tolerable, the patient comfort, the patient anatomical features, the presence of some obstructed vein and local preferences.
The size and position of the drainage cannula are the main determinants of ECBF. For ECBF higher than 3–4 l/min, drainage cannula size to up 23–28 Fr is necessary. The best position is the intrahepatic portion of the inferior vena cava or in the right atrium. Cannulas with multiple holes distributed along the cannulas are available to enhance blood drainage (multiple-stage drainage cannulas). The reimmission cannulas normally have holes only in a short portion near to their extremity.
For VV-ECMO four different cannula configurations are available (Fig. 7.2):
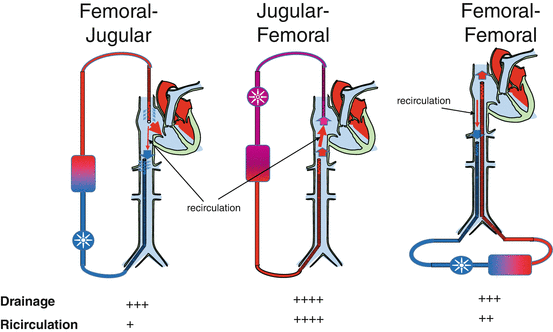
- 1.
Femoral-jugular configuration: The drainage cannula (21–28 Fr, 38–55 cm long) is inserted through the femoral vein and positioned with the tip in the inferior vena cava, while the reimmission cannula (19–21 Fr, 15–38 cm long) is inserted through the jugular vein with the tip in the right atrium. This represents the most used and also the easiest configuration. ECBF rates up to 6–7 L/min are easily obtained with minimal recirculation [16].
- 2.
Femoro-femoral configuration: The drainage cannula (21–28 Fr, 38–55 cm long)is inserted through the femoral vein and positioned with the tip in the inferior vena cava, while the reimmission cannula (21–23 Fr, 50–55 cm long) is inserted through the contralateral femoral vein with the tip positioned in the inferior vena cava (but higher than the drainage cannula) or in the right atrium. The femoro-femoral approach offers safer access and less possibility of accidental cannula dislocation. Mobilization of the patient’s head is facilitated, but at the expense of lower limb mobilization. With this approach, minimization of recirculation requires careful positioning of the cannula tips.
- 3.
Jugular-femoral configuration: The drainage cannula (21–25 Fr, 15–38 cm long) is inserted through the jugular vein and positioned with the tip in the right atrium, while the reimmission cannula (19–23 Fr, 15–55 cm long) is inserted through the femoral vein. Though popular in some centre, this approach is not much diffused given the high recirculation.
- 4.
Single-vessel double-lumen cannula: A single double-lumen cannula is inserted through the jugular vein. Different types of double-lumen cannulas are available. The most popular is the AVALON ELITE® Bi-Caval Dual Lumen Catheter [17–20]: the cannula is positioned through the jugular vein with the tip in the inferior vena cava. The configuration of the cannula allows drainage of blood from both the superior and inferior vena cava, while reimmission is in the right atrium. Sizes ranging from 13 to 31 Fr are available.
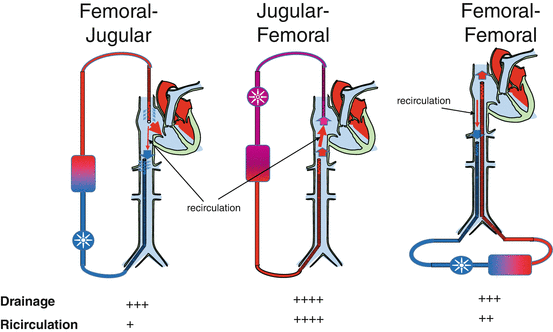
Fig. 7.2
A schematic of cannula configuration using single-lumen cannulas. Independently from the reimmission cannula, the drainage cannula should be placed with the tip above renal veins. Drainage performance and recirculation rate are reported on the bottom. Though drainage is considered easier with the jugular-femoral approach, recirculation is higher and the effective extracorporeal blood flow is lower than with the other configurations
7.2.4 Anticoagulation
The contact between patient blood and the ECMO foreign surface is associated with activation of coagulation factors and complement factors, platelets and fibrinogen consumption [21–24], which may lead to bleeding [25] and thromboembolic [26] complications. For these reasons, continuous anticoagulation is necessary throughout the ECMO treatment. Anticoagulation during VV-ECMO is commonly achieved by continuous intravenous heparin infusion, targeted to a partial thromboplastin time (aPTT) of 45–60 s and/or to an activated clotting time (ACT) of 1.5–2 times normal. Transfusion policy regarding platelet count varies among centres. Transfusion thresholds between 50,000 and 100,000/μL have been reported. Activation of the coagulation cascade and clot formation in the circuit are accompanied by a progressive decrease in platelet count and fibrinogen level and consumption of coagulation factors that may lead to a syndrome similar to disseminated intravascular coagulation. For this reason, activation of the coagulation and circuit thrombosis, along with oxygenator performance and/or increase of transmembrane pressure, must be monitored to understand the need for a prompt replacement of ECMO circuit. D-dimer levels have been shown to be a reliable variable to detect circuit thrombosis.
7.3 ECMO for ARDS: A Historical Perspective
Long-term applications of ECMO support are an evolution of heart-lung machine use in cardiac surgery. The first successful use of prolonged ECMO in an adult ARDS patient was reported by Hill et al. in 1972 [27]. After only 2 years from first successful cases, the National Institute of Health commissioned a multicentre randomized clinical trial on prolonged ECMO for adults with ARDS [28]. At the time, the main indication for ECMO in ARDS patients was to correct the hypoxaemia while buying time for the lungs to heal [29]. As such, high ECMO blood flow rates were necessary to improve oxygenation, and the veno-arterial configuration was the standard. Following the negative results of the study, which failed to show any survival benefit from ECMO, only few investigators continued studying and improving the technique. Contrary to adult applications, applications in newborn showed encouraging results [30–35]. This contributed to maintain some interest on ECMO.
A landmark contribution to the evolution of adult ECMO came from the pioneering work done in the laboratory of T. Kolobow at the NIH. In a series of study, Kolobow and Gattinoni showed that [36–40] (1) nearly all the metabolic CO2 production could be removed through an artificial lung using much lower extracorporeal blood flows than those required to oxygenate the blood and (2) removal of CO2 through the membrane lung allows to reduce, virtually to zero, ventilation of the native lung. Based on these observations, they developed the concept of extracorporeal CO2 removal (ECCO2R) and proposed the use of a VV bypass configuration instead of the classical VA mode and the use of low-frequency ventilation to allow lung rest [40]. Unfortunately, a single-centre, randomized controlled trial conducted by Morris et al. in 40 ARDS patients failed to show a benefit from this technique [41]. Nevertheless, few centres around the world continued to apply ECMO in adult patients, contributing to improve the ECMO technique for long-term applications [42–45].
Following the concept of lung rest developed by Kolobow et al., several animal and human studies have demonstrated that, though necessary to preserve life, mechanical ventilation (MV) can exacerbate lung damage eventually contributing to mortality [46–54]. This has led to the concept of ventilator-induced lung injury (VILI) [54] and of “protective ventilatory strategy”, mainly consisting of low Vt (6–8 mL/Kg PBW) and limitation of plateau airway pressures (Pplat <28–30 cmH2O). ECMO is a unique technique to master protective ventilation and lung rest since, as Kolobow foresaw, it allows to minimize the need for mechanical ventilation allowing for using extremely low tidal volumes and to apply safe plateau pressures even in the most severe patients. Finally, in 2009, Peek et al. published the results of the Conventional Ventilation or ECMO for Severe Adult Respiratory Failure (CESAR) trial [55]. The study enrolled 180 adult patients (18–65 years) with severe but potentially reversible acute respiratory failure, defined as a Murray score ≥3 or uncompensated hypercapnia with a pH < 7.20. Patients randomized to receive ECMO were transferred to the Leicester ECMO centre, while controls remained in designated treatment centres. The trial showed a survival advantage (47% vs 63% at 6 months) associated with the use of ECMO in adults. Unfortunately, the enthusiasm for the positive results has been mitigated by important limitations of the study. First, not all patients allocated to the ECMO group received ECMO, because they died before or during transportation (five patients) or they improved with conventional treatment after transportation to the ECMO centre (17 patients). Second, as there was no standardized protocol for mechanical ventilation in the control group, significantly fewer patients in the control group received a protective ventilatory strategy. However, despite these limitations, the results of the study have led to an increase in interest in ECMO worldwide. Incidentally, publication of the study almost coincided with the outbreak of H1N1 influenza in 2009. Following the successful use of ECMO in patients with H1N1-induced severe ARDS reported from the Australian and New Zealand experience [50], several countries recurred to the use of ECMO, even organizing national network able to centralize the most severe patients. The published case series from these experiences report survival rates ranging from 68 to 83% [56–63].
Since 2009, the use of respiratory ECMO in adults has continued to grow. The relative simplification of the technique together with the acquisition of experience has push more and more centres to institute specialized ECMO teams able to employ different ECMO techniques for different indications.
7.4 Indications to VV-ECMO
There are two main indications to VV-ECMO in ARDS (Fig. 7.3):
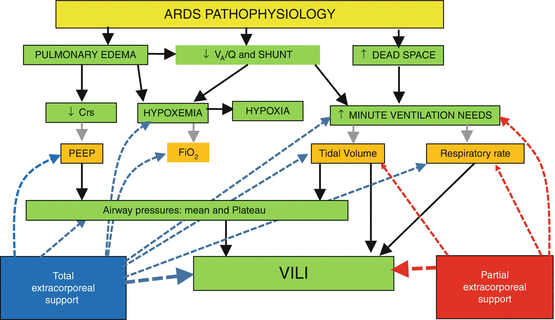
- 1.
Refractory hypoxia: This is the classical indication. The aim is to deliver oxygen and improve oxygenation in patients with hypoxaemia unresponsive to other treatments. For this indication, VV-ECMO is employed with high ECBF that provide both oxygen delivery with improvement of oxygenation and CO2 removal to decrease tidal volume and plateau pressure reducing the burden of injurious mechanical ventilation. This VV-ECMO application is generally identified as total extracorporeal support.
- 2.
Injurious ventilation: In some patient nearly acceptable blood gas values can be obtained but at the expense of high minute ventilation with high tidal volumes/respiratory rates and high inspiratory plateau pressures. Given the higher efficiency of VV-ECMO in removing CO2, decrease of minute ventilation, tidal volume, respiratory rate and consequently airway pressures can be obtained with relatively low ECBF (<2 l/min). When CO2 removal is the main indication and VV-ECMO is employed at relatively low ECBF, we talk of partial extracorporeal support.
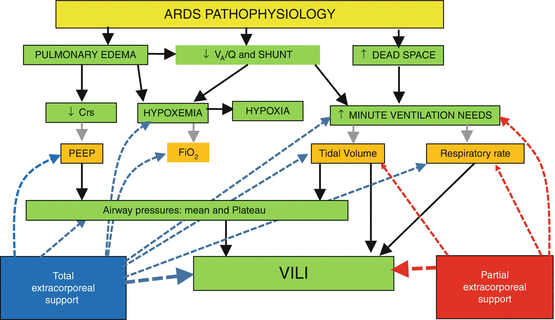
Fig. 7.3
A schematic of different pathophysiological effects of total and partial extracorporeal support. Main pathophysiological alteration of ARDS (green boxes) and therapeutic implications (orange boxes) are reported. Total extracorporeal support allows to relieve hypoxia (and consequently decrease FiO2 and PEEP requirements) and to minimize ventilator-induced lung injury (VILI) (allowing to decrease tidal volume, respiratory rate and consequently airway pressures). Partial extracorporeal support is indicated only to minimize VILI as it does not have any positive effect on patient oxygenation. V A /Q ventilation perfusion ratio, SHUNT intrapulmonary shunt, Crs respiratory system compliance
7.5 Total Respiratory Extracorporeal Support
Total respiratory extracorporeal support refers to ECMO application in the most severe acute respiratory failure patients with hypoxaemia refractory to lung-protective ventilation and adjunctive therapies like inhaled nitric oxide, prone positioning and recruitment manoeuvres. The aim is to provide viable oxygenation when the natural lung becomes unable to provide it and prevent the mechanical ventilation-associated lung damage. Therefore, total extracorporeal respiratory support is viewed as a rescue therapy for severely hypoxaemic ARDS patients.
The reason to institute ECMO in severe ARDS is to break the “vicious cycle” of tissue hypoxia (Fig. 7.3) [64]. Refractory hypoxaemia leads in the most severe cases to tissue hypoxia; in this setting the weapons that conventional mechanical ventilation uses to relieve hypoxaemia are the increase of FiO2 up to 1 and the increase of mean airway pressure (PEEP, I:E ratio manipulation, plateau, recruitment manoeuvres). The increase in intrathoracic pressures leads in turn to haemodynamic compromise defacing, in the most severe cases, the effects of the relief of hypoxaemia on tissue hypoxia. In this setting, institution of high-flow total ECMO support can provide viable delivery of oxygen to the tissues also when gas exchange through the natural lung is completely abolished.
Indeed, high-flow ECMO provides also total CO2 removal, abolishing the need of tidal ventilation of the natural lung to remove CO2. During ECMO the natural lungs can be maintained “at rest” avoiding high plateau pressures and high minute ventilation requirements that stretch the low-compliance “baby lungs” of severe ARDS leading to barotrauma, volutrauma and biotrauma and breaking therefore the vicious cycle of ventilator-associated lung injury (VALI or VILI) and the final dismal result of multiple organ failures (MOFs) leading to death [65].
7.5.1 Technical Requirements
To achieve the aim of viable oxygen delivery in severe ARDS patients, a high-flow, low-recirculation ECMO system is required. Both VA and VV-ECMO configurations can be used.
In the early 1970s, at the dawn of ECMO application in severe ARDS [28], the VA approach was used to achieve maximum oxygenation. VA-ECMO is characterized by high blood flow and null recirculation and therefore is an efficient system to oxygenate the blood, but in the setting of severe lung injury, its use has been abandoned due to the high rate of complications related to the arterial cannulation (bleeding, compartment syndrome, embolization) and the development of differential hypoxia (Harlequin syndrome) if the arterial cannula is not positioned centrally at the level of the aortic arch. Nowadays, the percutaneous VV configuration is used in more than 70% of severe refractory hypoxaemic patients, as reported by data from the Extracorporeal Life Support Organization (ELSO) registry [66, 67].
ECBF as stated before is the main determinant of oxygenation during total respiratory ECMO support. Reported ECBF in the refractory hypoxaemic patients ranges from greater than 3–6 l/min. The amount of cardiac output (CO) oxygenated by the artificial lung, i.e. the ECBF indexed to CO (ECBF/CO), must be greater than 60% to obtain an arterial O2 saturation >90% or a PaO2 >60 mmHg [68].
Large-bore drainage cannulas are a prerequisite for achieving the goal of a high ECBF in the setting of refractory ARDS. The other important factor is their location in relation to the position of the return cannula (Fig. 7.2). If the drainage and return cannula are in the inferior vena cava, through a femoro-femoral approach, the distance between the tip of the two cannulas is critical to minimize recirculation. The best VV configuration in terms of effective ECBF (total ECBF-recirculated ECBF) is the femoro-jugular approach. In contrast, the jugulo-femoral approach is the least efficient in terms of recirculation, followed by the femoro-femoral one. In the recent years the use of a single-vessel approach gathered the favours of the ECMO community; a double-lumen large-bore cannula (AVALON ELITE® Bi-Caval Dual Lumen Catheter) is inserted percutaneously in the internal jugular vein and drains blood from both inferior and superior vena cava if correctly positioned. The inner return cannula has a hole that is directed towards the tricuspid valves, minimizing recirculation of oxygenated blood [69].
7.5.2 What Is Refractory Hypoxaemia? Who Are the Candidates for Rescue ECMO Support?
Refractory hypoxaemia is reported as a cause of death in only 10–15% of ARDS patients, being multiple organ failure and sepsis the leading causes in the majority of the cases [70].
Esan et al. [71] defined refractory hypoxaemia as PaO2/FiO2 ratio <100 mmHg or inability to maintain Pplat <30 cmH2O despite a tidal volume of 4 mL/kg IBW or the development of barotrauma or an oxygenation index >30. According to the Berlin definition of ARDS [72], the candidates for ECMO as a rescue therapy are in the most severe category group characterized by PaO2/FiO2 <100 mmHg with PEEP ≥5 cmH2O. In the recently published LUNG SAFE study [73], ECMO was used as a rescue therapy in 48 out of 729 patients with severe ARDS (6.6%) in the 4 weeks enrolment period; assuming that the main reason for ECMO in this group was refractory hypoxaemia, the figure is slightly lower than the 10–15% incidence reported previously and can be explained by the more widespread use in the last 10 years of adjunctive manoeuvres and the adherence to lung-protective mechanical ventilation.
For the ELSO guidelines [74] ECMO “should be considered in hypoxic respiratory failure due to any cause (primary or secondary) when the risk of mortality is 50% or greater (PaO2/FiO2 <150 mmHg on FiO2 >90% and/or Murray score 2-3), and is indicated when the risk of mortality is 80% or greater (PaO2/FiO2 <100 mmHg on FiO2 >90% and/or Murray score 3-4 despite optimal care for 6 h or more)”.
In Table 7.1 we report the main criteria of selection reported in some clinical series and in published and ongoing ECMO trials [28, 41, 55, 56, 60–63, 75–78, 80], whereas in Table 7.2 the real pre-ECMO baseline data are reported from the largest published series in the last 10 years [55, 56, 60–63, 66, 68, 76–79, 81–86].
Table 7.1
Reported indications/contraindications for total extracorporeal respiratory support in ARDS
References | ECMO indications | ECMO contraindications |
---|---|---|
Zapol [28] Morris [41] | Fast entry: PaO2 <50 mmHg with FiO2 1.0 and PEEP ≥ 5 cmH2O Slow entry: PaO2 <50 mmHg for >12 h with FiO2 0.6 and PEEP ≥ 5 cmH2O with shunt fraction >30% | |
Linden [75] | Fast entry: PaO2/FiO2 <60 and shunt fraction >30% on FiO2 >0.9 for 2 h and diffuse infiltrates in four quadrants Slow entry: unresponsive to prone positioning, iNO and HFO; persistent hypercapnia | Age >60 Advanced MOFs Underlying severe disease Severe immunosuppression |
Hemmila [76] | PaO2/FiO2 <100 on FiO2 of 1.0, alveolar-arterial gradient (AaDO2) >600 mmHg, or shunt fraction >30% despite and after optimal treatment | Age >70 |
Peek [55] | Severe, potentially reversible ARF, Murray Lung Injury score (LIS) ≥ 3·0, or uncompensated hypercapnia with pH < 7·20 despite optimum conventional treatment | High pressure (peak inspiratory pressure >30 cmH2O) or high FiO2 (>0.8) ventilation >7 days Signs of intracranial bleeding Any other contraindication to limited heparinization Any contraindication to continuation of active treatment |
ANZ ECMO [56] | Refractory hypoxaemia (PaO2/FiO2 <60 mmHg), or hypercarbia (PaCO2 >100 mmHg, with PaO2/FiO2 <100) | Significant pre-existing comorbidities Weight >120 kg Pulmonary hypertension Cardiac arrest |
Roch [62] | PaO2/FiO2 <70 mmHg for at least 2 h at FiO2 1 and PEEP level adjusted to obtain Pplat 30 cmH2O, or PaO2/FiO2 <100 mmHg with Pplat >35 cmH2O, or respiratory acidosis with pH ≤7.15 despite RR ≥35/min | |
Patroniti [61] | At least one of the following criteria: OI >30 PaO2/FiO2 <70 with PEEP ≥ 15 cmH2O (patient already admitted to an ECMOnet centre); PaO2/FiO2 <100 with PEEP ≥10 cmH2O (patients still to be transferred) pH <7.25 for at least 2 h Haemodynamic instability | Absolute: Intracranial bleeding or other major contraindication to anticoagulation Previous severe disability Poor prognosis because of the underlying disease Relative: 1. MV >7 days |
Noah [60] | CESAR criteria for transfer ECMO instituted if adequate gas exchange could not be achieved with conventional lung-protective ventilation | |
Schmid [77] | PaO2/FiO2 ≤ 80 mmHg with FiO2 of 1.0, PEEP 18 cmH2O and refractory respiratory acidosis (pH ≤7.25), despite optimization of conservative therapy | |
Lindskov [78] | Before 2010: acute potentially reversible and potentially fatal respiratory failure Fast entry and slow entry criteria as reported by Zapol After 2010: PaO2/FiO2 <100 mmHg on FiO2 >0.9 and LIS 3–4 PaCO2 >100 mmHg because of asthma or permissive hypercapnia (plateau ≤ 30 cmH2O) Severe air leak syndromes | Before 2010: Mechanical ventilation >5 days and FiO2 1.0 >3 days Cancer Nonreversible CNS injury Chronic disease with short life expectancy Profound uncontrollable sepsis After 2010: There are no absolute contraindications to ECLS. Each patient is considered individually with respect to risks and benefits |
Pham [63] | Severe ARDS defined as: LIS >3 Arterial pH less than 7.21 PaO2/FiO2 <100 mmHg Arterial oxygen saturation <90% | |
Lehle [79] | Severe ARDS with LIS 3–4 and PaO2/FiO2 <80 mmHg on high PEEP (generally >15 cmH2O) despite optimization Relative indication for vvECMO: LIS 2–3 and a PaO2/FiO2 <150 mmHg Severe respiratory acidosis (pH < 7.25) Inspiratory pressure over 30 cmH2O Severe air leaks | |
EOLIA trial [80] | PaO2/FiO2 <50 mmHg with FiO2 >0.8 for >3 h, despite optimization of mechanical ventilation (Vt 6 mL/kg and PEEP >10 cmH2O) and despite adjunctive therapies (NO, recruitment manoeuvres, prone position, HFO ventilation, almitrine infusion) | Intubation and mechanical ventilation >7 days Pregnancy Weight >1 kg/cm or BMI >45 kg/m2 |
Table 7.2
Baseline characteristics and outcome of severe ARDS reported in large VV-ECMO series published in the last 10 years
References | n. pts | Age years | PaO2/FiO2 mmHg | PaCO2 | pH | PEEP cmH2O | PIP cmH2O | Plateau cmH2O | Pre-ECMO MV (days) | LIS | SOFA | ECMO days | Survival % |
---|---|---|---|---|---|---|---|---|---|---|---|---|---|
Schmidt [68] | 2355 | 41 (28–54) | 59 (48–75) | 56 (44–73) | 7.3 (7.2–7.4) | 13 (10–16) | 36 (31–43) | – | 2.4 (0.8–6.3) | – | – | 7 (4–13) | 57 |
Brogan [66] | 1473 | 35 (22–53) | 57 (45–71) | – | 7.3 (7.2–7.4) | 13 (10–16) | 40 (35–48) | – | 2.2 (0.8–6.7) | – | – | 23 ± 20 | 50 |
Lehle [79] | 317 | 50 (37–62) | 64 (51–79) | 7.2 (7.1–7.3) | 3.3 (3.3–3.7) | 9 (6–15) | 62 | ||||||
Enger [81] | 304 | 46 (43–48) | 69 (65–74) | – | 7.2 (7.2–7.3) | 16 (16–17) | 35 (34–36) | – | 5 (4–7) | 3.5 (3.4–3.5) | 11 (11–12) | 10 (9–11) | 59 |
Hemmila [76] | 280 | 38 ± 13 | 55 ± 16 | – | 7.3 ± 0.1 | 13 ± 5 | 44 ± 11 | – | 4 ± 3 | – | – | 9 ± 8 | 52 |
Schmid [77] | 176 | 48.0 ± 16.7 | 77 ± 47 | 70 ± 30 | 7.2 ± 0.2 | 18 ± 6 | 35 ± 6 | – | 6.1 ± 10.2 | 3.4 ± 0.5 | 12.3 ± 3.8 | 12 ± 9 (1–67) | 56 |
Schmidt [82] | 140 | 44 (30–56) | 53 (43–60) | 63 (51–77) | 7.2 (7.2–7.3) | 10 (8–12) | – | 32 (30–35) | 5 (1–11) | – | 12 (10–15) | 15 (8–30) | 60 |
Lindskov [78] | 124 | 42 (16–67) | 48 (37–60) | 57 | 7.26 ± 0.15 | – | – | 37 (35–41) | – | 3.7 | 13 | 9 (1–23) | 71 |
Noah [60] | 123 | 34 (28–46) | 54.9 ± 14.3 | – | – | – | – | – | – | – | 9.1 ± 2.9 | – | 73 |
Roch [62] | 85 | 47 ± 15 | 60 (50–70) | 59 (50–73) | 7.1 ± 0.2 | – | – | 32 (29–35) | 2 (1–8) | 3.5 (3.3–3.7) | 9 (7–11) | 9 (7–13) | 44 |
Rega [83] | 70 | 43 ± 18 | 56 ± 18 | – | 7.22 ± 0.18 | 13 ± 3 | 44 ± 11 | – | 4.5 ± 7.4 | – | – | 7 ± 5 | 43 |
Pham [63]
![]() Stay updated, free articles. Join our Telegram channel![]() Full access? Get Clinical Tree![]() ![]() ![]() |