CHAPTER 83 OXYGEN TRANSPORT
Unicellular organisms evolved to efficiently harness large amounts of energy from organic molecules (especially glucose) through the utilization of oxygen as an electron acceptor. In this process, CO2 is generated and chemical energy is transferred to high-energy containing molecules of ATP. Oxygen cannot be stored in our cells; therefore, the generation of energy through aerobic (oxygen consuming) processes is completely dependent on its supply. Without oxygen, death rapidly ensues.
In unicellular organisms, the oxygen consumed and the CO2 produced easily diffuses across the cell membrane. This cannot occur in multicellular organisms such as humans. Instead, complex mechanisms of oxygen delivery and CO2 removal had to evolve in order to maintain aerobic metabolism. We are therefore totally reliant on the coordinated function of respiratory, cardiovascular, and hematologic systems to maintain energy production.1
At a molecular-cellular level, shock is defined as the pathologic state that occurs when oxygen supply becomes the rate-limiting step in the generation of energy.2 We can now measure the degree of failure in the supply and utilization of oxygen during shock, and in fact using these variables, can monitor the effectiveness of therapy.3 This chapter intimately explains how we can quantify the delivery and consumption of oxygen.
ENERGY GENERATION IN THE CELL
Glycolysis (the first step in the process of glucose metabolism) requires the breakdown of glucose to two molecules of pyruvate. This first step occurs universally in the cytoplasm of all mammalian cells, yielding two molecules of ATP. This constitutes only 5.2% of the total potential energy that can be released from glucose. In the absence of oxygen, pyruvate is metabolized by lactic dehydrogenase to lactate. Under anaerobic conditions, such as that seen in intense physical activity or during shock, lactate rapidly accumulates in the circulation. Lactate uptake with regeneration of glucose occurs in the liver. Lactate concentrations in blood of less than 2 mmol/l are considered normal. Increased lactate production, poor lactate clearance, and accumulation in plasma reflect increased anaerobic metabolism and a state of shock. The degree of increase in plasma lactate is a reliable prognostic sign.4 Lactate levels can also be used as an endpoint of therapy in trauma resuscitation so that successful treatments of shock should be followed by normalization of lactate in plasma.5
In the aerobic portion of glucose metabolism, 36 molecules of ATP are generated. Thus, cellular respiration yields 18 times more energy than anaerobic glycolysis. Most of the cells in our body are dependent on cellular respiration for the generation of energy and ultimate survival. Organic molecules that generate pyruvate are not limited to glucose since other molecules such as certain amino acids and fatty acids can be utilized. It is therefore oxygen, not pyruvate, which becomes the rate-limiting step in the generation of energy in our bodies.6
Pathologic states that involve abnormal mitochondrial utilization of oxygen are observed in various clinical processes. For example, cyanide poisoning of the electron transfer chain results in a rapid development of a severe energy deficit, accumulation of lactate, and death. More commonly, states of septic shock are thought to cause “mitochondrial disease” that renders these organelles incapable of efficiently utilizing oxygen for the generation of energy.7 To date, physicians can do little to correct mitochondrial dysfunction.
MICROCIRCULATION AND OXYGEN DELIVERY
A breakdown in the regulation of oxygen distribution (dysoxia) across the microcirculation occurs in septic shock; thus, septic shock is a form of distributive shock. Excessive amounts of nitric oxide, a potent vasodilator, are thought to be a central aspect of sepsis dysoxia. In addition, the resultant tissue edema increases the distance between the cells and the capillaries, which also contributes to poor oxygen diffusion. Thus, sepsis is characterized by poor oxygen extraction.8
Hemoglobin, the Ultimate Oxygen Carrier
Hemoglobin (Hb) serves as a unique oxygen carrier capturing oxygen in the alveoli, distributing it through the microcirculation, and ultimately discharging it in the pericellular environment. Each gram of hemoglobin binds 1.34 ml of O2. Since Hb concentration is easily measured, the content of O2 carried per 100 ml of blood can be calculated as follows7,9:
Example:
The degree of oxygen saturation in Hb through spectrophotometric absorption of light (pulse oximeter) is a widely used tool in intensive care. The saturation of hemoglobin is highly nonlinear, roughly following an S curve (Figure 1). This determines that Hb can easily upload or download O2 under physiologic conditions. It also determines that minor drops in SaO2 at saturations of less than 90% reflect higher degrees in the change of the partial pressures of O2 (PO2) than drops at a lower SaO2. Roughly, a 90% SaO2 reflects a PO2 of 60 mm Hg. Pathologic alterations of the Hb-oxygen saturation curve are observed with alterations in pH, temperature, and the concentration of 2-3 diphosphoglycerate (2-3 DPG).
< div class='tao-gold-member'>
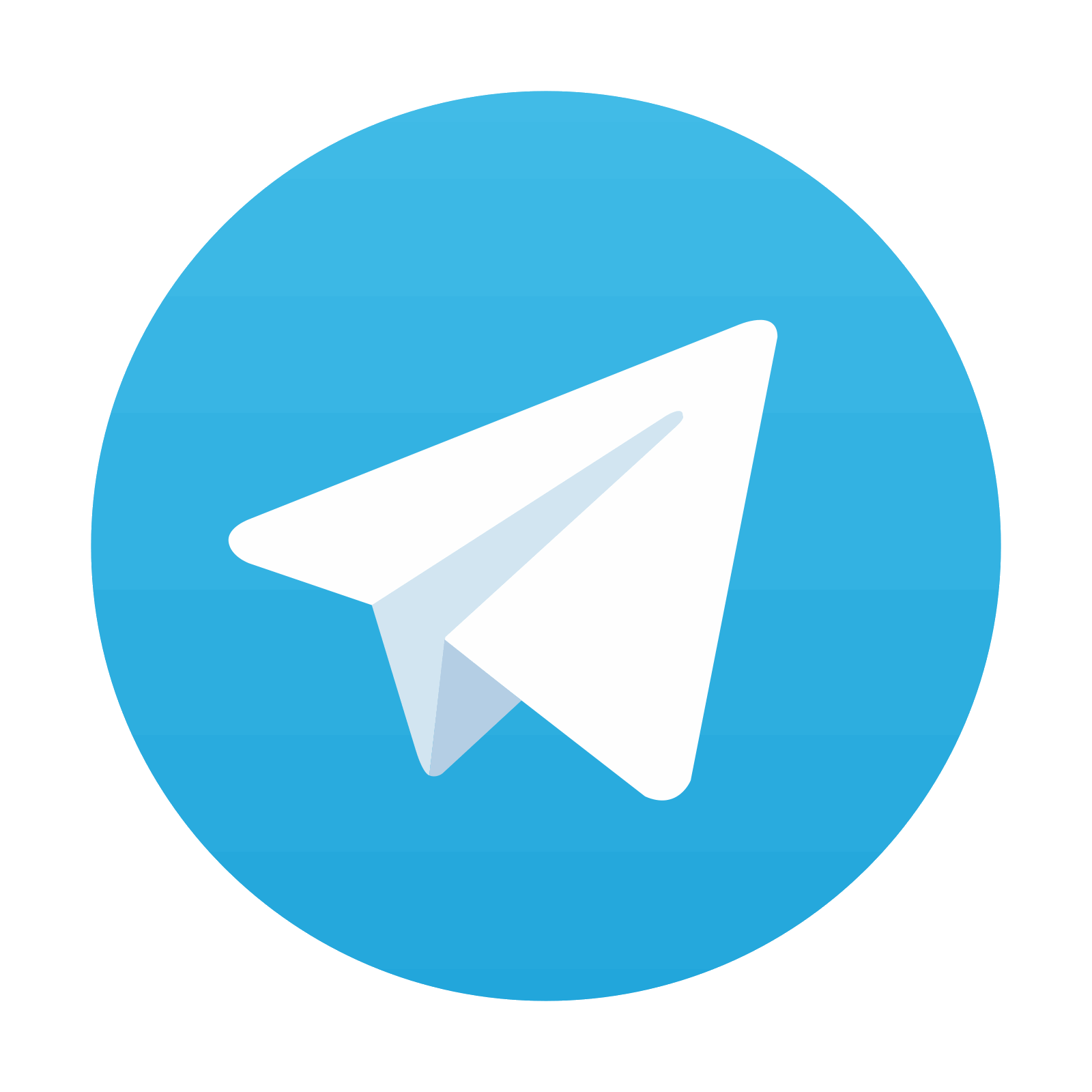
Stay updated, free articles. Join our Telegram channel
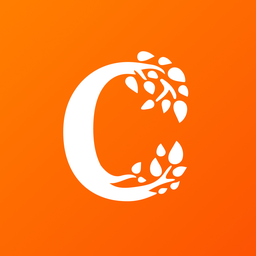
Full access? Get Clinical Tree
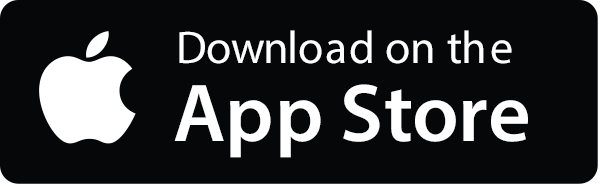
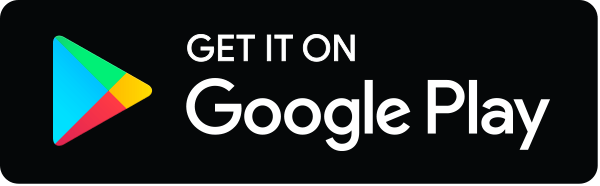