See text for explanations of points a, b, c, and d. PAW = airway pressure; Pes = esophageal pressure.
With the pressure breath, neither volume nor flow is being set. The inspiratory pressure in the circuit is simply being set. In this case, the dependent variable is flow and volume. If patient effort increases, the volume and flow will go up. If effort decreases, the reverse happens. If compliance or resistance improves, the flow and volume will also increase. If the compliance or resistance worsens, the flow and volume will decrease. When a patient is using these breaths, the flow and volume should be monitored because those are what will change.
There are 3 common types of breath cycles. The breath reaches a set volume (ie, a volume-cycled breath), a set time (ie, a time-cycled breath), or a certain flow reduction (ie, a flow-cycled breath). This gets a little confusing because airway pressure usually functions as a backup cycle, which means that if the pressure exceeds certain limits, usually under the condition of an airway occlusion or active exhalation, the breath will cycle off to prevent overpressurization. Newer ventilators have a pressure-release mechanism, so that if pressure starts to increase because the patient starts to forcefully exhale, for example, then instead of the breath turning off, the machine will allow the patient to exhale, thus providing relief. This type of breath forms the basis of airway pressure release ventilation (APRV). This pressure release breath is very similar to a pressure-controlled breath, except for the fact that during the delivery of gas, the patient can breathe spontaneously because of the release mechanism. Table 1 illustrates the 7 basic breaths available on all ventilators.
Table 1. Breath characteristics.
Modes of Support
Six common modes of ventilation are available. Volume-assist-control is a mixture of volume-control and volume-assist breaths. If the rate is very high, it is pure volume-control. If the rate is very low, letting the patient breathe, it is pure volume-assist. The same principles apply to pressure-assist-control, except that pressure-targeted breaths are being used. If the rate is very high, it is in the pressure-controlled mode. If the rate is very low, it is using pressure-assist breaths. Volume cycled synchronized intermittent mandatory ventilation (SIMV) mixes volume-assist-controlled breaths with pressure-supported or just pure spontaneous breaths. Pressure SIMV mixes pressure-assist-controlled breaths with either pressure support or spontaneous breathing. The APRV mode usually employs long inspiratory times and functions like pressure SIMV with spontaneous breaths (or pressure support breaths) occurring during both inspiration and expiration. Pressure support can also function as a stand-alone mode.
Newer ventilators have feedback-control mechanisms, the most common of which is called pressure-regulated volume control. This control adjusts the pressure target to maintain a target tidal volume. It combines variable flow with volume target, which may improve patient ventilator synchrony. However, it will not limit pressure in the setting of worsening mechanics. This feedback control principle can also be used as a pressure-support mode as well, in which case it is sometimes called volume support. Another newer type of feedback system is called adaptive support ventilation. Basically this algorithm adjusts the tidal volume and rate to allow for the ventilator to do the least amount of work, which in theory minimizes the inspiratory strain on the lungs. One concern, however, is that ASV may drive the tidal volume slightly higher than clinicians may be comfortable with (eg, >6mL/kg) Importantly, none of these feedback mechanisms has been validated in any large clinical trials in terms of outcome improvement.
Basic Respiratory System Mechanics
Simply described, the respiratory system consists of the tracheobronchial tree and 300 million alveoli enclosed by the thoracic cage. Pressures are usually measured in the ventilator circuit (often referred to as airway pressure). This pressure is determined by gas flow and volume interacting with airway resistance and respiratory system compliance. By stopping flow, this circuit pressure equilibrates with alveolar pressure. This stop flow is commonly done at end inspiration and the resulting circuit pressure (alveolar pressure) is known as the plateau pressure, so named because when airway pressure becomes flat, it plateaus. When the stop flow is used during expiration, the clinician can determine what alveolar pressure is during expiration. When this is done at end expiration, the alveolar pressure reflects intrinsic positive end-expiratory pressure (PEEPi). The pleural pressure can also be estimated with the use of an esophageal balloon. Although the latter is not commonly used, esophageal balloons are not difficult to place for those who have access to them, and they can provide some interesting physiologic information.
Pressure, Flow, and Volume
Mechanical effects of positive-pressure ventilation can be explained as follows: the ventilator applies positive airway pressure, causing the alveolus to expand and push the chest wall outward. As a result, the pleural pressure becomes positive.
In Figure 2, the inspiratory flow is 1 L/s. The expiratory flow is 2 L/s. The volume tracing is 1 L. The peak pressure is a combination of the pressures required for flow and pushing the lung and chest wall out. In this case, the peak pressure is 40 cm H2O. When a stop flow is applied, the flow-related pressures disappear (10 cm H2O). The plateau pressure, which reflects the pressure to distend the lung and chest wall, is thus 30 cm H2O. The pleural pressure (estimated by esophageal pressure) increases from 0 to 10 cm H2O. This is the pressure required to push the chest wall out of the way. If it takes 30 cm H2O (eg, the plateau pressure) to push out both the lung and chest wall, and it takes 10 cm H2O to do just the chest wall, then it takes 20 cm H2O to push the lung alone.
Figure 2. Mechanical effects of positive-pressure ventilation.
During a spontaneous breath, the inspiratory muscles are pulling the chest wall out and the lung open. Consequently, the pleural pressure is now negative. In fact, if the flow and volume delivery are similar, it resembles an upside-down airway pressure from a ventilator controlled breath. That is because respiratory system mechanics are the same, regardless of whether the patient is pulling the gas in or the ventilator is pushing the gas in. With similar flow and an end-inspiratory pause, the same mechanics calculation can be done to determine the various pressures.
If this patient develops flash pulmonary edema, the lung will stiffen. It will not affect the flow or the chest wall. There will be high peak and plateau pressures, but the pleural pressure will not change. So this elevation in plateau pressure reflects an increase in lung stiffness, not chest wall stiffness.
Interestingly, a stiff chest wall—not a stiff lung—can be responsible for driving up the plateau pressure. Anasarca, ascites, obesity, or chest bandages could be the cause of low chest wall compliance. Putting the patient in the prone position can also cause a bit of this effect. What is important to recognize is that the pressures are being driven up by the chest wall. Measuring pleural (esophageal) pressure is the only way to actually quantify the respective roles of lung vs chest wall compliance on the plateau pressure.
Ventilator Induced Lung Injury
There is compelling evidence that the lung can be injured when either excessive stretch or repetitive alveolar collapse-reopening occurs during mechanical ventilation (ventilator induced lung injury or VILI). The concept of providing “lung protective” ventilatory strategies thus embodies limiting tidal volume and plateau pressure while also providing appropriate positive end-expiratory pressure (PEEP). In practice this usually means targeting tidal volumes of 6-8 mL/kg ideal body weight, keeping plateau pressures < 30 cm H2O, and providing PEEP levels that result in adequate oxygenation.
A static pressure volume plot of the respiratory system may be a guide to optimizing these settings – the goal being to place the lung on the deflation limb of the curve well below the overdistention point and well above the re-collapse point. Unfortunately, static pressure volume plots are very time consuming and difficult to do. Many ventilators today, however, do what is called a slow-flow maneuver, which the clinician can use to approximate a static pressure–volume curve. When flow is delivered slowly, the pressures required for flow becomes small. This makes the dynamic single breath curve approximate the static pressure–volume curve.
Air Trapping
The phenomenon known as air trapping, or PEEPi, is caused by 3 things: high minute ventilation, long I:E ratio, and long expiratory time constants. Air trapping can cause many problems. For example, if the patient is receiving volume-targeted ventilation and starts to build up PEEPi, all the pressures will start to rise. If the patient is receiving pressure-targeted ventilation and starts to build up PEEPi, all the volumes will start to fall. Although PEEPi can be sneaky, the expiratory flow is a clue that something is going wrong. When there is inadequate time for complete lung emptying between breaths, the expiratory flow does not return to baseline. Monitoring the expiratory time will help ensure that it is adequate for the flow to return to the baseline.
Another way of detecting and measuring PEEPi is known as an expiratory pause, or a hold. Just as during inspiration with the plateau pressure, the lung is put under no-flow conditions at end expiration and the circuit pressure is measured. This has to be done under controlled mechanical ventilation, however, because patients will start to make breathing efforts and confound the measurement.
Using ventilator settings, PEEPi can be fixed by decreasing the minute ventilation, regardless of whether it is frequency or tidal volume, shortening the I:E ratio. Improving lung mechanics can also reduce PEEPi.
It should also be noted that PEEPi can serve as a substantial inspiratory threshold load with assisted breaths. This can be quantified by analyzing pleural (esophageal) pressure during patient triggering efforts. The classic sign of this threshold load is a considerable pleural (esophageal) pressure swing with a markedly delayed circuit pressure change (or even no change at all). These pleural (esophageal) pressure signals can also be used to guide the application of PEEP in the ventilator circuit to equilibrate with the PEEPi and thereby reduce the threshold load.
Mechanical ventilators are both simple and complex. Knowing the principles of mechanical ventilation, such as the design features, breath delivery, and modes of support, will enable clinicians to better understand what they are delivering to their patients, why the patients are responding in certain ways, and what key things to look out for, such as VILI risks and PEEPi.
Self-Assessment
- A ventilator breath that is patient-triggered, pressure-targeted, and time-cycled is called which of the following?
(a) Volume-assist breath
(b) Pressure-support breath
(c) Pressure-control breath
(d) Pressure-assist breath
- The plateau pressure is affected by all of the following EXCEPT:
(a) Inspiratory flow
(b) Chest wall compliance
(c) Lung compliance
(d) Positive end-expiratory pressure (PEEP)
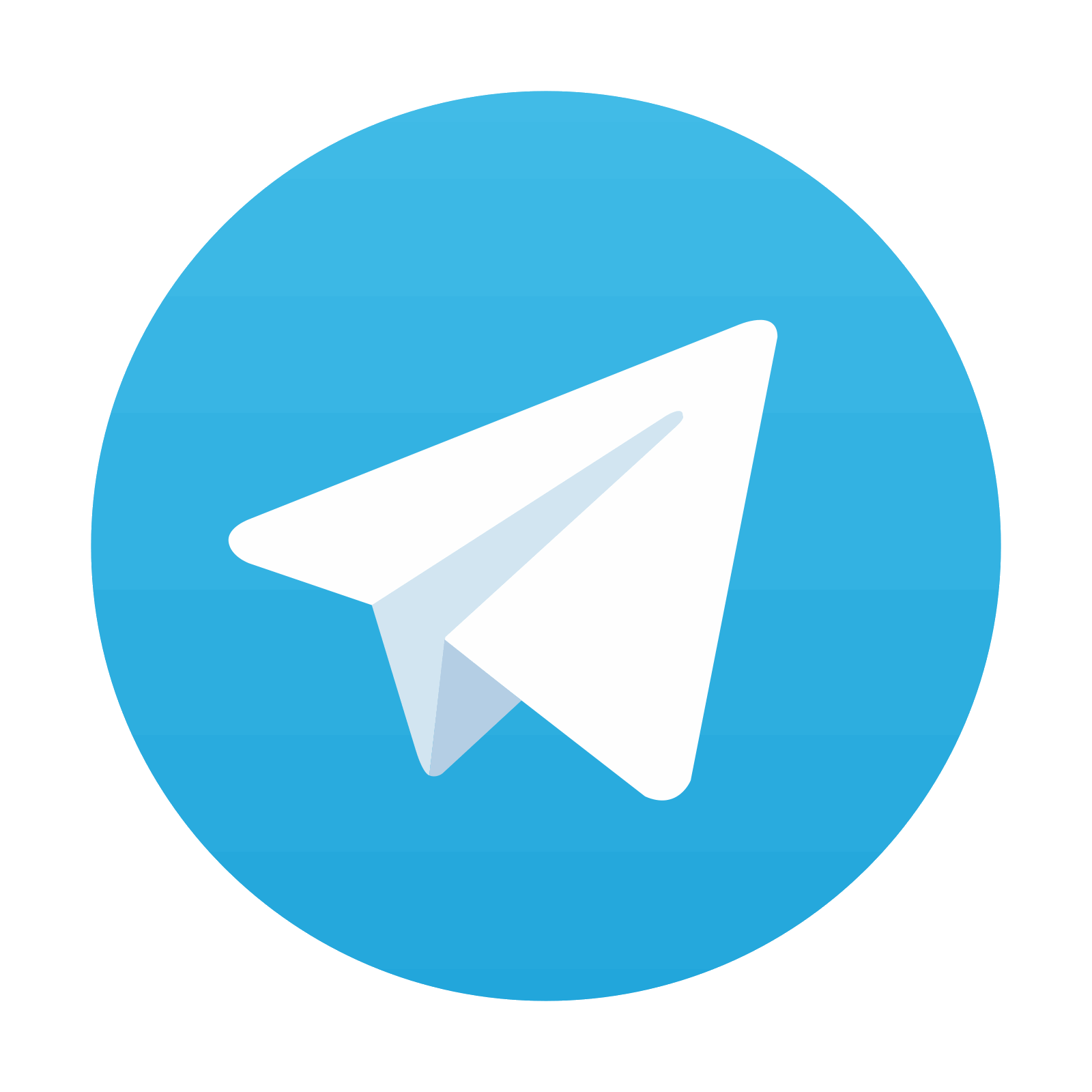
Stay updated, free articles. Join our Telegram channel
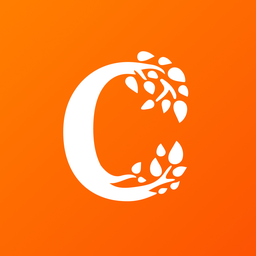
Full access? Get Clinical Tree
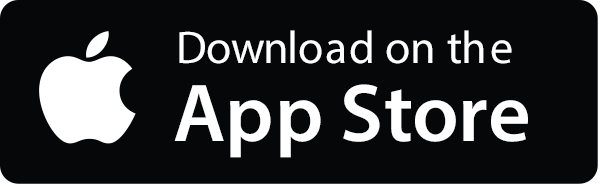
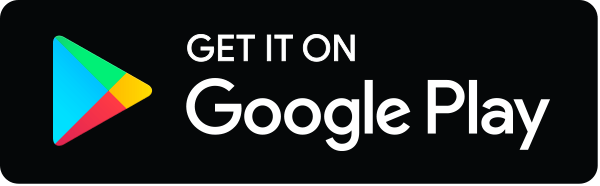