Chapter 75 Nutrient Metabolism and Nutrition Therapy During Critical Illness
Malnutrition is prevalent in critically ill children at the time of admission to the pediatric intensive care unit (PICU).1,2 Further nutritional deficiencies during the course of their illness are often incurred due to the burden of illness or suboptimal nutritient intake and may result in poor outcomes. Safe provision of optimal nutrients during hospitalization is an important goal of pediatric critical care. The changing metabolic state during the course of critical ilness results in unpredictable energy demands that need to be carefully matched with evidence-based nutritional strategies. However, prediction, estimation, and measurement of true energy expenditure in PICU patients can be challenging. Failure to accurately estimate or measure energy expenditure can potentially result in unintended underfeeding or overfeeding. While underfeeding has long been recognized as a problem, a significant proportion of critically ill children are at the risk of being overfed.3 Furthermore, there exist a myriad of barriers that impede the delivery of prescribed nutrients to the critically ill child and result in a delay or a failure to achieve the prescribed energy goal. The complexities of critical care or the nature of illness frequently conflict with nutrient provision. However, many barriers to bedside nutrient delivery may be avoidable. Examination of existing literature, audits of bedside practice, and multidisciplinary collaborations have helped identify optimal nutritional strategies, illuminated areas of practice deficiencies or knowledge gaps, and highlighted future priorities for research. There has been a resurgence in awareness and an increase in our understanding of the role of nutrition therapy during pediatric critical illness. However, the perceived benefits of novel therapies such as immunonutrition, tight glycemic control, and hormonal modulation of the stress response have not yet been realized in the general PICU population. Future studies will clarify the role of these strategies in improving patient outcomes in the PICU. Until then, careful screening for malnutrition, awareness of the metabolic state during the course of illness, accurate assessment of energy demands with attention to energy balance, multidisciplinary efforts to overcome common barriers to nutrient intake at the bedside, and a commitment to prioritizing nutritional support during critical illness are necessary to meet nutritional goals.
Malnutrition in the Critically Ill Pediatric Patient
Critical illness increases metabolic demand on the host in the early stages of the stress response, when nutrient intake may be limited. As a result, children admitted to the PICU are at risk of deteriorating nutritional status and anthropometric changes with increased morbidity.1 This effect is more pronounced in a subgroup of patients who are already malnourished or at risk of malnutrition on admission. The prevalence of malnutrition in children admitted to the ICU has remained largely unchanged over the last two decades. One in every four children admitted to the PICU shows signs of acute and/or chronic malnutrition on admission.1,2 A majority of PICU patients present with conditions that impede normal growth. Nutritional status affects physiologic responses and influences outcome. Malnutrition is associated with increased physiologic instability and the need for increased quantity of care in the ICU.4 Despite its high prevalence and consequences, medical awareness of malnutrition is lacking. The nutritional status of hospitalized patients is not routinely assessed, and only a minority of patients are referred for expert nutritional consultation or support.5 Careful nutritional evaluation at admission to the PICU will allow identification of children at risk for further nutritional deterioration and, hence, candidates for interventions to optimize nutrient intake.
Assessment of Nutritional Status
Assessment of the nutritional status in the critically ill child is vital but often challenging. Clinicians use a combination of anthropometric and laboratory data to diagnose undernourishment. Carefully elicited past history with details of weight gain, dietary history, recent illness, and medications allows identification of risk factors for preoperative malnutrition. Weight on admission to the hospital is important and may be the only measure of the actual dry weight before capillary leak syndrome results in edema and weight gain. Unless regular and accurate weights are obtained, acute changes in nutritional status may be missed or detected late.6 Children in the PICU are often not weighed as the procedure is deemed to be unsafe or not important. The lack of availability of reliable weight trends in PICU patients reflects the overall low priority among health care workers for nutritional assessment and, as a result, the true incidence of malnutrition in this cohort may indeed be underestimated. Physical examination should be directed toward specific signs of nutritional and metabolic deficiencies. Hair, skin, eyes, mouth, and extremities may reveal stigmata of protein-energy malnutrition or vitamin and mineral deficiencies.
A variety of other measurements including arm anthropometry (mid-upper arm circumference and triceps skin fold), body length, and body mass index have been used to monitor growth in children. In a study of infants and children admitted to the PICU, significant anthropometric abnormalities were detected by changes in mid-arm circumference and weight in correlation with energy deficits.1 These anthropometric abnormalities accrued during the PICU admission returned to normal by 6 months after discharge. Using reproducible anthropometric measures, other investigators detected malnutrition in a majority of children on admission to their PICU.7 Children with malnutrition had increased mortality compared with those without malnutrition.7 On follow-up, a significant portion of children with malnutrition had further deterioration in nutritional status. Although bedside anthropometric methods are inexpensive, they are sporadically applied in hospitalized children, may be insensitive in the setting of critical illness, and are limited by significant interobserver variability. Weight changes and other anthropometric measurements in critically ill children should be interpreted in the context of edema, fluid therapy, volume overload, and diuresis. In the presence of ascites or edema, ongoing loss of lean body mass may not be evident using weight monitoring alone.
Body Composition
Body composition is emerging as a primary determinant of health and a predictor of morbidity and mortality in children. Preservation and accrual of lean body mass during illness are important predictors of clinical outcomes in patients with sepsis, cystic fibrosis, and malnutrition.8,9 Body composition is measured by a variety of techniques including body densitometry by underwater weighing, neutron activation analysis, total-body potassium determination, bioelectrical impedance assessment (BIA), and dual-energy x-ray absorptiometry (DXA). Most of these methods are not practical for application in the clinical management of a critically ill child. DXA is a radiographic technique that can determine the composition and density of different body compartments (fat, lean tissue, fat-free mass, and bone mineral content) and their distribution in the body. DXA has been used extensively in pediatric practice for determining fat-free mass, fat mass, and lean mass, and it is recognized as a reference method for body composition research.10 Its results correlate well with direct chemical analyses, and there is good agreement between percentage body fat estimated by hydrodensitometry and DXA.11 However, DXA is not practical for application in the PICU. BIA, in contrast, is a bedside technique that can be applied to pediatric patients without exposure to radiation and with ease.12,13 Electrical current is conducted by body water and is impeded by other body components. BIA estimates the volumes of body compartments, including extracellular water and total body water (TBW). TBW measures can be used to estimate lean body mass by applying age-appropriate hydration factors. BIA has not been validated in critically ill populations; hence, its use outside clinical studies is not recommended in the PICU. The ideal bedside body composition measurement technique in critically ill patients remains elusive.
Biochemical Assessment
The nutritional status can also be assessed by measuring the visceral (or constitutive) protein pool, the acute-phase protein pool, nitrogen balance, and resting energy expenditure (REE). Visceral proteins are rapid-turnover proteins produced in the liver. Low circulating levels of visceral protein are seen in the setting of malnutrition, inflammatory states, and impaired hepatic synthetic function. The reliability of serum albumin as a marker of visceral protein status is questionable. Albumin has a large pool and a half-life of 14 to 20 days, and is not an indicator of the immediate nutritional status. Serum albumin may be affected by changes in fluid status, albumin infusion, sepsis, trauma, and liver disease, and these changes are independent of nutritional status. Prealbumin (also known as transthyretin or thyroxine-binding prealbumin) is a stable circulating glycoprotein synthesized in the liver. It binds with retinol-binding protein and is involved in the transport of thyroxine and retinol. Prealbumin, so named by its proximity to albumin on an electrophoretic strip, is readily measured in most hospitals and is a good marker for the visceral protein pool.14,15 It has a half-life of 24 to 48 hours and reflects more acute nutritional changes. Prealbumin concentration is diminished in liver disease. Acute-phase reactant proteins are elevated proportional to the severity of injury in response to cytokines released during stress response and have been used to longitudinally monitor the inflammatory response. Serum levels of acute-phase protein are elevated in children within 12 to 24 hours after burn injury, due to hepatic reprioritization of protein synthesis.16 When measured serially, serum prealbumin and C-reactive protein (CRP) are inversely related (i.e., serum prealbumin levels decrease and CRP levels increase, with the magnitude proportional to injury severity, and then return to normal as the acute injury response resolves). In infants after surgery, decreases in serum CRP values to less than 2 mg/dL have been associated with the return of anabolic metabolism and are followed by increases in serum prealbumin levels.17 Proinflammatory cytokines such as interleukin 6 (IL-6) are recognized as early markers of the systemic inflammatory response syndrome (SIRS) in several disease models. Serum concentrations of IL-6 may be useful in identifying patients at risk for nutritional deterioration and to determine whether the inflammatory response is intact.
Nutritional Requirements During Critical Illness
Metabolic Consequences of the Stress Response
Importantly, nutritional support itself cannot reverse or prevent the metabolic stress response. Failure to provide optimal calories and protein during the acute stage of illness can result in an exaggeration of existing nutritional deficiencies or further exacerbate an underlying poor nutritional status. Respiratory compromise involving loss of respiratory muscle mass, cardiac dysfunction and arrhythmias involving loss of myocardial muscle tissue, and intestinal dysfunction involving loss of the gut barrier contribute to the morbidity and mortality of critical illness. In some cases, overestimation of this energy cost of metabolic stress may result in provision of energy in excess of requirement. Hence, large energy imbalances attributable to underfeeding and overfeeding in critically ill children must be avoided.3 This requires an individualized nutritional regimen that must be tailored for each child and reviewed regularly during the course of illness. A basic understanding of the metabolic events that accompany critical illness and surgery is essential for planning appropriate nutritional support in critically ill children.
The unique hormonal and cytokine profile manifested during critical illness is characterized by an elevation in serum levels of insulin, glucagon, cortisol, catecholamines, and proinflammatory cytokines.18 Increased serum counterregulatory hormone concentrations induce insulin and growth hormone resistance, resulting in the catabolism of endogenous stores of protein, carbohydrate, and fat to provide essential substrate intermediates and energy necessary to support maintenance energy and micronutrient needs in addition to the ongoing metabolic stress response. Figure 75-1 illustrates the basic pathways involved in the metabolic stress response.
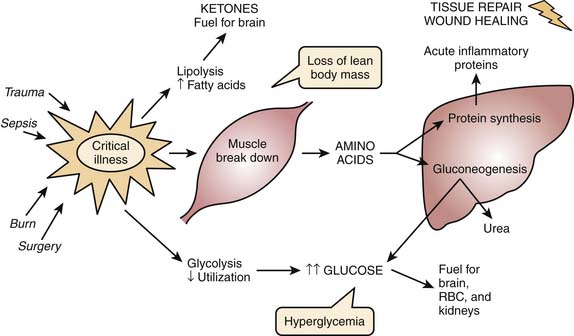
Figure 75–1 The metabolic response to stress.
(Modified from Mehta N, Jaksic T: The critically ill child. In: Duggan C, Watkins JB, Walker WA, editors: Nutrition in pediatrics, Hamilton, ON, 2008, BC Decker.)
In general, the net increase in muscle protein degradation, characteristic of the metabolic stress response, results in a large amount of free amino acids in the circulation. Free amino acids are used as the building blocks for the rapid synthesis of proteins that act as inflammatory response mediators and are used for tissue repair. Protein breakdown may continue for an extended period of time, in an attempt to channel the amino acids through the liver, wherein their carbon skeletons are used to create glucose through gluconeogenesis and for production of glucose as the preferred energy substrate for the brain, erythrocytes, and renal medulla. Reprioritization of protein during the metabolic stress results in increased synthesis of acute-phase reactant proteins such as C-reactive protein, α1-acid glycoprotein, haptoglobin, α1-antitrypsin, α2-macroglobulin, ceruloplasmin, and fibrinogen. Plasma concentrations of other proteins, including transferrin and albumin, decrease with injury or sepsis. Overall intense protein catabolism outstrips anabolism with a net negative protein balance. This condition results in weight reduction and rapid loss of lean body mass. The intense catabolism seen in metabolic stress cannot be suppressed by supplying calories, and negative protein balance continues relentlessly. This is one of the principal differences between stress response and starvation. Starvation, or protein-calorie malnutrition, may be caused by socioeconomic, psychosocial, disease-related, or iatrogenic factors. The metabolic response to starvation involves decreased secretion of insulin and thyroid hormones, normal secretion of glucocorticoids and catecholamines, and decreased oxygen consumption. In starvation states, the body tries to preserve itself by using less energy for basic metabolic functions; thus, overall metabolic rate decreases. Metabolism shifts to use fat as a primary energy source, and the corresponding ketones help provide fuel for the brain and spare glucose and protein utilization. However, body tissues still must be broken down to supply amino acids for other critical functions, eventually leading to loss of lean body mass and vital organ wasting, and possibly death. Although, provision of additional proteins does not suppress protein catabolism, it may decrease the negative protein balance by increasing protein synthesis. Table 75-1 summarizes the basic differences between starvation and metabolic stress.
Table 75–1 Metabolic Stress vs. Starvation
Metabolic Stress | Starvation | |
---|---|---|
BMR | ↑↑ | ↔↓ |
Oxygen consumption (VO2) | ↑↑ | ↓ |
Protein catabolism | ↑↑↑ | ↔ |
UUN | ↑↑ | ↔ |
Weight loss | Rapid | Slow |
LBM loss | Early | Late |
Response to caloric intake | Protein catabolism continues | Protein catabolism halted |
Insulin, cortisol, and catecholamines | ↑↑ | ↓ |
Ketones | ↑↑ | ↔ |
Gluconeogenesis | ↑ | ↓ |
BMR, Basal metabolic rate; UUN, urinary urea nitrogen; LBM, lean body mass.
Supply of adequate nutritional intake under these circumstances is challenging, and yet recovery of critically ill patients depends on their ability to utilize energy substrates and synthesize new proteins. Carbohydrate turnover is simultaneously increased during the metabolic response, with a significant increase in glucose oxidation and gluconeogenesis. The administration of exogenous glucose does not blunt the elevated rates of gluconeogenesis, however, and net protein catabolism continues unabated.19 A combination of dietary glucose and protein may improve protein balance during critical illness, primarily by enhancing protein synthesis. The stress response to injury stimulates lipolysis and increased rates of fatty acid oxidation.20 Increased fat oxidation reflects the premiere role of fatty acids as an energy source during critical illness. Triglycerides in adipose tissue are then cleaved by hormone-sensitive lipase into fatty acids and glycerol. Fatty acids are oxidized in the liver for energy via the tricarboxylic acid or Krebs cycle. As seen with the other catabolic changes associated with stress response, the provision of dietary glucose does not decrease fatty acid turnover in times of illness. The increased demand for lipid use in the setting of limited lipid stores puts the metabolically stressed neonate or previously malnourished child at high risk for the development of essential fatty acid deficiency.21,22 Preterm infants are most at risk for developing essential fatty acid deficiency after a short period of a fat-free nutritional regimen.22,23 The beneficial effects of the acute metabolic response to illness/injury must be considered in relation to the harmful consequences of a persistently severe catabolic response. Nutritional therapy should aim to support the metabolic changes occurring during the acute catabolic stage. With resolution of a hypermetabolic stress response, an anabolic phase typically follows, with increased release of GH and IGF-1. Supply of adequate nutrition is essential for this recovery phase. In summary, the metabolic response to critical illness results in glucose and lipid intolerance and increased protein breakdown.
Underfeeding and Overfeeding in the Pediatric Intensive Care Unit
Individual assessment of energy requirements and provision of optimal nutritional support should be the standard of care. Both underfeeding and overfeeding are prevalent in the PICU, with resultant nutritional deficiencies that are associated with complications.4,24 True energy expenditure during acute illness may not be easily predicted and several studies have documented discrepancies in measured versus equation-estimated energy expenditure.25–27 Children with severe burn injury demonstrate extreme hypermetabolism in the early stages of injury. Standard equations have been shown to underestimate the measured REE in this population.28 Unless increased energy requirements during the acute stage of such illnesses are accurately measured and matched by adequate intake, cumulative energy deficits will ensue with decrease in weight, loss of critical lean body mass, and a worsening of existing malnutrition. Physicians have reported significantly escalated energy demands in a child with severe paroxysmal dysautonomia associated with ischemic brain injury. Failure to estimate this increased energy need resulted in underfeeding in this patient with severe weight loss during the course of illness in the PICU.6 A variety of barriers, both unavoidable as well as some avoidable, exist that impede optimal nutrient delivery at the bedside and contribute to the likelihood of underfeeding in the PICU.29,30 In the setting of fluid shifts, edema, and capillary leak in acute illness, some of these negative anthropometric outcomes may not be detected by the existing crude assessment techniques. Underfeeding during acute illness, with cumulative negative energy balance, has been associated with poor outcomes in critically ill adults.31 However, energy imbalance in of the PICU population may also present in the form of cumulative energy excess due to unintended overfeeding. Indeed overfeeding in the PICU may be an underrecognized entity with a potential impact on patient outcomes.
Children do not predictably mount the characteristic hypermetabolic stress response as is seen in adults. The metabolic response to stress from injury, surgery, or illness is variable and the degree of hypermetabolism is unpredictable and unlikely to be sustained during a prolonged course in the PICU.32 Critically ill children cannot be presumed to be hypermetabolic following acute illness or injury and energy expenditure may actually be decreased in some groups of patients.33,34 While a sustained increase in metabolism has been reported for weeks after burn injury, REE peak returns to baseline within 12 hours after some surgical procedures.35,36 Indeed children on extracorporeal life support or after surgery have failed to show any significant hypermetabolism, and measured energy expenditure is close to resting energy expenditure in these populations.37 Critically ill children who are sedated and mechanically ventilated may have significant reduction in actual total energy expenditure, due to multiple factors. Decreased activity during illness, attenuation of insensible fluid losses in the controlled PICU environment, and transient absence of growth during the acute illness all keep total REE close to the basal rate, even in critically ill children. Historically, stress or activity correction factors have been traditionally factored into basal energy requirement estimates to adjust for the nature of illness, its severity, and the activity level of hospitalized subjects.38,39 These patients may be at a risk of overfeeding when estimates of energy requirements are based on age-appropriate equations developed for healthy children, and especially if stress factors are incorporated in an attempt to account for the perceived hypermetabolic effects of the illness. Indirect calorimetry testing to determine the true metabolic state must be considered before incorporating stress factor correction to energy estimates in critically ill children. While the problems with underfeeding have been well documented, overfeeding too has deleterious consequences.24,40 Overfeeding increases ventilatory work by increasing carbon dioxide production and can potentially prolong the need for mechanical ventilation. Overfeeding may also impair liver function by inducing steatosis and cholestasis, and increase the risk of infection secondary to hyperglycemia. There are no data in general pediatric populations for the role of hypocaloric feeding.41 In general, the energy goals should be assessed and reviewed regularly in critically ill children.
Assessing Energy Expenditure in Critically Ill Patients
Although the Food Agricultural Organization and the World Health Organization (WHO) have recommended that energy requirements and dietary recommendations be based on measurements of energy expenditure, the resources and expertise for such measurements are not easily available in all units. Current recommendations for nutritional requirements of the critically ill child are derived from limited data, based on studies in healthy children and based on limited methodologic approaches. Table 75-2 summarizes recommended energy and protein intake for critically ill children. Recommendations for pediatric nutritional requirements have traditionally focused on the supply of nutrients for growth. The components of total energy expenditure in children include (1) basal metabolic rate (BMR) 70%, (2) diet-induced thermogenesis (DIT) 10%, (3) energy expended during physical activity (PA) 20% and (4) energy expended for growth. The sum of these components determines the energy requirement for an individual. The traditional components of energy expenditure in healthy children may not apply during critical illness (see Table 75-3). Thus, prescribing optimal energy for the critically ill child requires careful review of each component of total energy expenditure.
Table 75–3 Components of Energy Expenditure: Normal Health vs. Critical Illness
Component | Normal Health | Critical Illness |
---|---|---|
BMR (60%–70%) | Energy needed for maintaining vital processes of the body Measured in a recumbent position, in a thermoneutral environment after 12–18 hours fast, when the individual has awakened before starting daily activities Not practical for bedside Sleeping energy expenditure, a component of BMR was shown to be equal to REE = 0.9 Corresponds to lean body mass | Related to metabolic state May be increased in conditions such as inflammation, fever, acute or chronic disease (e.g., cardiac, pulmonary) Related to lean body mass |
REE (50%–60%) | BMR + 10% Usually measured instead of BMR REE is measured at rest in a thermoneutral environment, after 8–12 hours fast and not immediately after awakening | Measured by indirect calorimetry with steady-state conditions |
DIT or TEF (10%) | Reflects the amount of energy needed for food digestion, absorption, and part of synthesis | Increased energy needs following enteral feeding return to baseline approximately >4 hours of feeding |
Growth (variable) | Energy for growth may be higher is healthy infants <2 years and during catch-up growth | Probably halted? |
PA (variable) | Depends on age, activity level Decreased in hospitalized patients | Sedation, muscle relaxants, decreased activity |
Stress | — | Variable Probably overestimated during critical illness |
Total energy expenditure | REE + DIT + PA + Growth | Probably close to REE in most critically ill children Addition of stress factors may be necessary where relevant |
BMR, Basal metaboic rate; REE, resting energy exenditure; DIT, diet-induced thermogenesis; TEF, thermic effect of food; PA, physical activity.
Previous recommendations for energy requirements were based on estimates of basal metabolic rate or REE derived by either indirect calorimetry or standard equations.34,42 Studies examining the performance of estimated energy needs in relation to measured REE in critically ill children are small-sized prospective or retrospective cohort studies. REE estimates have a large individual variability, and predictive equations are unreliable, particularly in underweight, overweight, or critically ill children.27,43,44 Newer equations have attempted to improve the prediction of REE in children by accounting for weight-based groups or by including pubertal staging, with variable success.44,45 These equations have not been satisfactorily validated in critically ill children.46 The variability of the metabolic state may be responsible for the failure of estimation equations in accurately predicting the measured REE in critically ill children. The application of stress factors might predispose some patients to the risk of overfeeding. Hence, it might be prudent to refrain from using these corrections in the absence of an accurate measurement of REE. Application of hypocaloric feeding has been recommended in critically ill adults.47 There is not enough evidence to recommend its general use in critically ill children.
Indirect Calorimetry
Historically, indirect calorimetry (IC) has been regarded as the gold standard for accurate measurement of REE. Energy expenditure is obtained by measuring the volume of oxygen consumed (VO2) and the volume of CO2 produced (VCO2) over a period of time.48 From this estimate the 24-hour energy intake is derived. Measurements of VO2 and VCO2 are used to calculate REE using the modified Weir equation: REE = [VO2 (3.941) + VCO2 (1.11)] × 1440. This technique has been validated in healthy children by using a whole-body chamber to allow 24-hour measurement. For obvious reasons, the whole-body chamber cannot be used in critically ill children.
The application of IC in different PICU populations has shown the variability in energy expended during illness. Weekes and Elia showed a relatively higher resting metabolic rate in critically ill children (37% higher than the resting metabolic rate of age-matched healthy controls).49 However, the total energy expenditure was reduced in a group of head-injured children receiving enteral nutrition. Energy expenditure was noted to decrease over time and returned to normal after the second week of injury. In critically ill mechanically ventilated children, use of sedation and muscle paralysis decreases the component of energy requirement related to physical activity,50 and caloric needs in the critically ill child may be lower than previously considered. IC continues to be only sporadically applied in critically ill children, despite mounting evidence of the inaccuracy of estimated basal metabolic rate using standard equations. This could potentially subject a subgroup of children in the PICU to the risk of underfeeding or overfeeding. However, IC application is not feasible in all patients due to (1) specific subject requirements, (2) device limitations, and (3) need for expertise and resources. Table 75-4 describes some of the common problems associated with IC testing in critically ill children. In the era of resource constraints, IC may be applied or targeted for certain high-risk groups in the PICU.3 Selective application of IC may allow many units to balance the need for accurate REE measurement and limited resources (see Box 75-1 for suggested criteria for targeted IC).51 While IC application has illuminated our understanding of energy expended during critical illness, this has yet to be translated into improving patient outcomes. Studies examining the role of simplified IC technique, its role in optimizing nutrient intake, its ability to prevent overfeeding or underfeeding in selected subjects, and the cost-benefit analyses of its application in the PICU are desirable. The effect of energy intake on outcomes needs to be examined in pediatric populations, especially in those on the extremes of body mass index (BMI).
Table 75–4 Factors Associated with Inaccurate or Unreliable Indirect Calorimetry Measurements
Error in VCO2 Measurement | Limitations or Mechanical Issues with the Device | Failure to Reach Steady State |
---|---|---|
Air leak >10% around endotracheal tube | High inspired FiO2 (>60%) | Recent interventions (suctioning, painful procedure) |
Air leak in the circuit | Calibration issues | Fever, seizures, dysautonomia |
Chest tube for pneumothorax | Moisture or obstruction due to water in the circuit | Recent change in ventilator settings |
Study period too short |
Box 75–1 Suggested Criteria for Targeted Indirect Calorimetry3,51
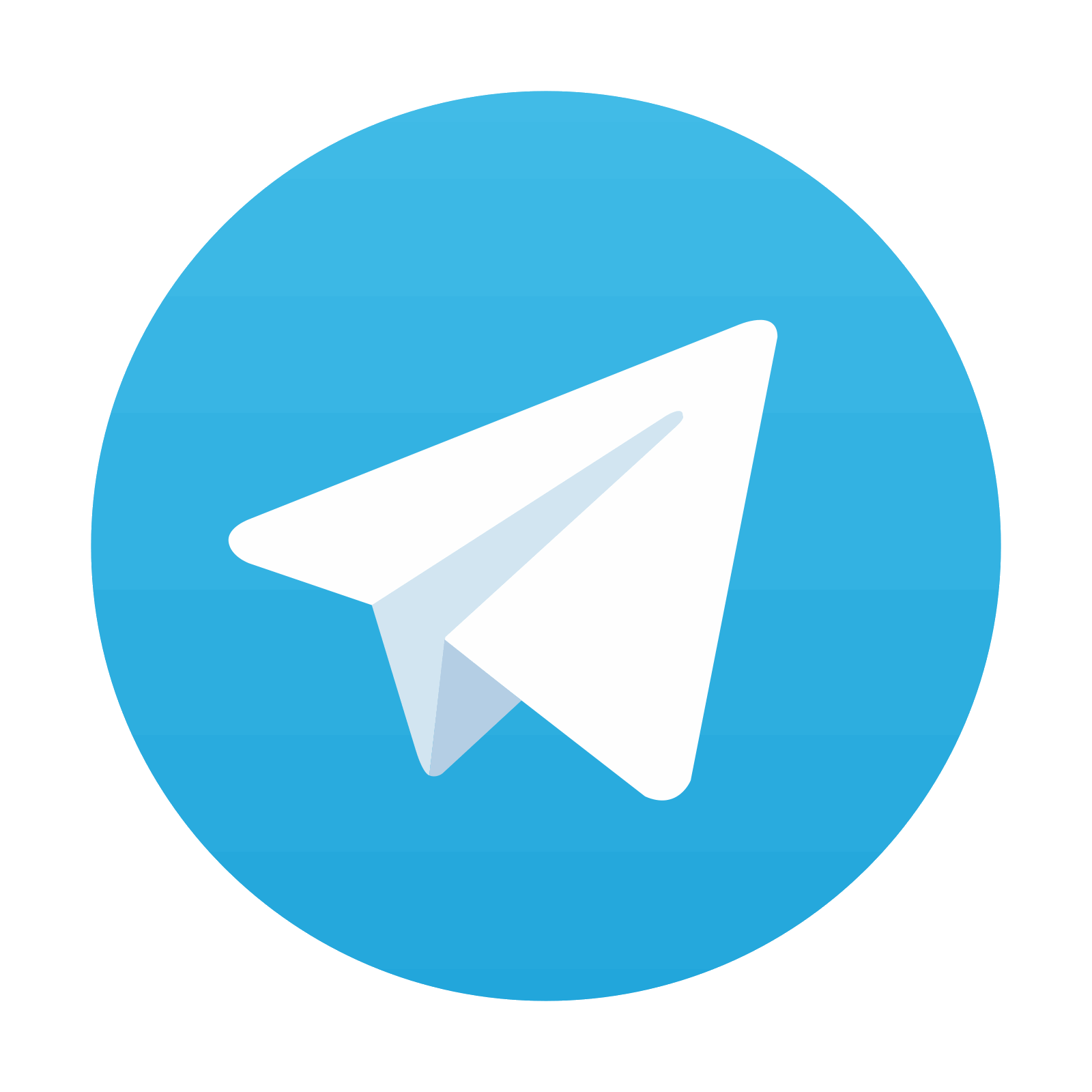
Stay updated, free articles. Join our Telegram channel
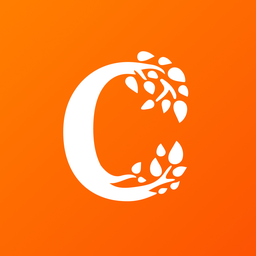
Full access? Get Clinical Tree
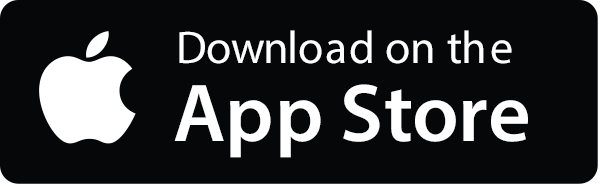
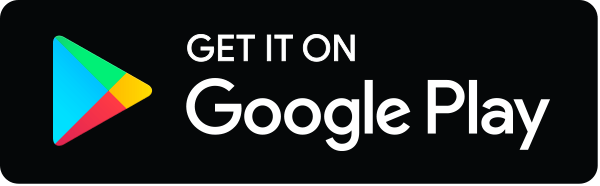