Chapter 43 Noninvasive Monitoring in Children
Noninvasive monitoring in the form of vital signs (i.e., heart rate, respiratory rate, noninvasive blood pressure, fluid intake and output, and temperature) has been used routinely for all patients receiving care in the intensive care unit (ICU) since the birth of the specialty. Guidelines for equipment and monitoring and for levels of care for pediatric ICUs (PICUs) were specified by the American College of Critical Care Medicine in 2004.1 It has become increasingly apparent that accurate and continuous core temperature management may significantly influence outcomes in pediatric critical care. Admission temperature is inversely related to both mortality and late-onset sepsis in low birth weight infants.2 Cold stress may lead to or worsen hypoglycemia in late preterm infants.3
Vital Signs
Blood Pressure Measurement
Accurate, continuous measurement of noninvasive blood pressure (NIBP) in infants and children can be challenging. Current methods of measuring NIBP are limited to auscultation, oscillometry, ultrasound, and the flush or return to flow.4 Because of technical difficulties, routine, reliable measurements of NIBP in infants and children did not become possible until approximately 4 decades ago. Auscultatory determinations, even on an intermittent basis, can be difficult to obtain in infants. In the flush or return to flow technique, the distal extremity is compressed, facilitating blood drainage. An occluding cuff is inflated proximally on the limb and gradually deflated. The systolic pressure is the pressure at which flow returns to the compressed distal extremity.
Numerous subjective influences play a major role in accuracy. Sensitive sound amplification systems have been used, but not commonly in the ICU setting. The most popular method used is based on the principle of oscillometry (e.g., Dinamap, Critikon, Tampa, Fla.), which automatically inflates and deflates the cuff and uses crystal microphones and piezoelectric crystals and the Doppler principle to measure and display systolic, mean, and diastolic pressures. During oscillometry, blood flow through an artery during cuff deflation causes the arterial wall to oscillate.5 The rapid increase in oscillation amplitude represents systolic pressure, whereas the sudden decrease in oscillation represents diastolic pressure. The period of maximum oscillation is used to estimate mean blood pressure.
The needle bounce technique is another oscillometric method commonly practiced by flight nurses/physicians. An inflated distal or proximal extremity circumferential cuff is slowly deflated, and the first visible bounce corresponds to systolic pressure. Automatically obtained blood pressures by the oscillometric method compare favorably with those obtained via arterial cannulas in infants and children.6–8 Oscillometric devices do not perform well if there is significant limb movement or in the presence of dysrhythmias.4 Occlusive systems using frequent measuring intervals can be associated with problems such as skin breakdown after prolonged use.4,9
Doppler devices are extremely useful for determining blood pressure in small babies, particularly when shock is present. First, a small Doppler probe is placed over an extremity artery. Blood movement causes changes in exquisitely sensitive ultrasound reflectance, and thus, as a cuff on the proximal extremity is slowly deflated, systolic pressure is signaled by the appearance of the first Doppler effect signal. Diastolic pressure is read when the strength and quality of the signal decrease. Correlation of these two pressures with arterial catheter pressures is good.10 However, it is important to place the Doppler probe directly over an artery. When an arterial catheter is not available and the automatic oscillometric device is not able to provide pressure readings, the Doppler technique can be used to obtain intermittent blood pressure measurements in the ICU setting.
When measuring blood pressure in babies and children, it is important to select the appropriate-sized blood pressure cuff. Numerous cuff sizes are available, including neonatal sizes. For the upper extremity, the cuff should occupy at least two thirds of the upper arm.11 The cuff bladder circumferential dimension should be 20% greater than that of the extremity.11 A cuff that is too small will result in falsely increased readings.7,11 In contrast, an oversized cuff will artificially decrease blood pressure readings, but the magnitude of this error is small.7
Temperature Monitoring and Routine Temperature Management
Body temperature can dramatically alter physiology and metabolism. Monitoring of temperature is a routine part of the practice in the ICU. The accepted normal range of rectal temperature in children is from 36.1° C to 37.8° C.12 This range is closely guarded by an intact thermoregulatory system that controls heat production and loss. However, many ICU patients can be considered poikilothermic, meaning they are unusually sensitive to environmental temperature fluctuations. A number of factors may contribute to this poikilothermic tendency, including the presence of either endogenous or exogenous vasoactive influences, hypothalamic dysfunction, the administration of drugs that blunt the normal regulation of body temperature, and depression of the central nervous system (CNS), either endogenous or exogenous from administration of sedatives at moderate to high doses. Temperature fluctuations in such patients may occur rapidly. For this reason, the continuous monitoring of core temperature can be particularly useful in selected patients, including those with increased intracranial pressure or status epilepticus who are managed with high-dose CNS depressants and mechanical ventilation; those with unstable hemodynamics after open heart surgery; those experiencing respiratory failure and extreme mechanical ventilation support; and, of course, those being observed for the development of malignant hyperthermia.13
Temperature Monitoring Sites
Because core temperature is the principal thermoregulatory controller, monitoring core temperature is more useful than monitoring peripheral skin temperature. Commonly used core temperature monitoring sites include the distal esophagus, tympanic membrane, pulmonary artery, and nasopharynx. These sites detect core temperature changes rapidly, in contrast to urinary bladder or rectal measurements, which are good reflections of core temperature during steady-state conditions.14 Cutaneous temperature monitoring is the least reliable indicator of rapid core temperature changes. However, monitoring peripheral temperatures can be useful in defining core peripheral gradients in temperature and assist in tracking vasoconstriction and vasodilation. Oral probes are used as thermometers, and some have been attached to pacifiers. A thermometer that scans the temporal artery is also available.15
Axillary and peripheral skin probably are the most convenient sites for monitoring temperatures, although they also provide the most inaccurate readings because of skin perfusion. These sites may be monitored through use of a wearable continuous-read precision phase change thermometer, which is a dot matrix system of heat-responsive indentations that is secured to the patient via an adhesive backing. In a dot matrix thermometer color changes occur in the dots at specific temperatures related to the melting point of the specific chemical materials in each dot.15 When continuous core temperature monitoring is desirable, the most reliable and convenient device is the lower esophageal temperature probe.
Pulse Oximetry
Takuo Aoyagi, working for the Nihon Kohden Corporation in Japan, first proposed the theory for pulse oximetry in 1972. His idea was developed into a working oximeter, which subsequently was patented in Japan in 1974 and marketed as the world’s first commercial pulse oximeter. In 1977 a fiberoptic-based pulse oximeter with improved accuracy was marketed by Minolta, and in 1982 Nellcor began marketing a pulse oximeter that ultimately became an industry standard.16 Since then numerous companies have produced and marketed pulse oximeters, and improvements in technology continue to improve the accuracy and reliability of these devices. Most recently, Masimo Corporation (Irvine, Calif.) introduced pulse oximeters with signal extraction technology17 that minimizes motion artifact and interference from ambient light and is able to function in relatively low perfusion states. This approach has improved the accuracy of pulse oximetry readings and has decreased the frequency of false alarms in clinical settings.18–22
There is also a “blue” sensor that is particularly sensitive in patients with cyanotic congenital heart disease.23 Standard pulse oximeters are not accurately calibrated for the low saturations seen with some congenital cardiac lesions. Accurate pulse oximetry is especially vital in the neonatal population, who benefit from tight control of oxygenation in order to minimize oxidative stress and to decrease the risk of retinopathy of prematurity.24
Principles of Pulse Oximetry
Pulse oximetry is based on the elegant observation that the attenuation of light passing through blood-perfused tissue changes with pulsation of blood and that the alternating component of the light attenuation results from the composition of arterial blood.25 Figure 43-1 is a schematic diagram showing that the component of light attenuation as a result of pulsatility comes from arterial blood. This information can be analyzed to determine the hemoglobin saturation in the arterial blood. Absorption of light as a result of other tissue components and capillary and venous blood in the static portion of the signal is ignored in the analysis.
where I is light emerging from the sample, Io is incident light illuminating the sample, ε is molar extinction coefficient of the specific absorbing species at a specific wavelength, b is path length (in centimeters) the light traverses, and c is molar concentration of the absorbing species. Thus changes in the concentration of an absorber, that is, oxyhemoglobin, results in changes in absorbance.
Hemoglobin has characteristic light-absorbing properties that change with oxygen binding. Figure 43-2 shows absorption spectra of oxyhemoglobin and deoxyhemoglobin in the visible and near-infrared spectral region. At any given wavelength there is a difference in absorption between oxyhemoglobin and deoxyhemoglobin except where the spectra cross at wavelengths called isosbestic wavelengths, where the absorption is the same for each state. At nonisosbestic wavelengths, the difference in absorption can be used to determine the fraction of oxyhemoglobin. Saturation of hemoglobin is defined as follows:
where Hbsat is fractional saturation of hemoglobin, [oxyHb] is concentration of oxyhemoglobin, and [deoxyHb] is concentration of deoxyhemoglobin. Hemoglobin percent saturation, as commonly reported, is determined by multiplying Hbsat by 100.
Pulse oximeters typically use two wavelengths of light to determine the saturation of hemoglobin, usually one around 660 nm in the visible light region and one around 940 nm in the near-infrared region.17 The absorption around 940 nm is relatively low and fairly constant over the range of saturations; thus a change in absorbance at 660 nm can be referenced to the absorption at the 940-nm wavelength and is used to determine the saturation. Each pulse oximeter uses a complex algorithm to convert the change in absorbance at the two wavelengths to an absolute saturation value. More wavelengths can be used to improve the accuracy of the measurement.
where [metHb] is concentration of methemoglobin and [carboxyHb] is concentration of carboxyhemoglobin. Most pulse oximeters usually cannot accurately account for the presence of these other forms of hemoglobin. Blood cooximeters, however, do account for these species, as do the newer Masimo pulse oximeters.
Validation
Numerous studies have been performed to validate existing pulse oximeters.26,27 Pulse oximeters also must be subjected to extensive testing prior to obtaining U.S. Food and Drug Administration (FDA) approval for marketing in the United States. Despite all of the current testing, difficulties in both calibration and validation remain. One of the most significant issues surrounding calibration is the development of an appropriate universal test that will accurately test the pulse oximeter for a wide range of potential clinical applications. Pulse oximeters must be accurate for a wide range of skin thickness and color and over a wide range of saturations. In general, pulse oximeters are most accurate at higher saturations, usually above 75%.28–30
Sources of Error
Although pulse oximetry is widely accepted as a valid clinical monitor and provides valuable minute-to-minute clinical data, pulse oximeters are subject to multiple potential sources of error. The most clinically significant source of error usually results from movement or “motion artifact,” which, as most clinicians recognize, results in frequent and annoying false alarms. Other sources of error include dyshemoglobinopathies, interfering dyes or other pigments in the blood, ambient light,31 and poor tissue perfusion. The extent of ambient light interference has been questioned for some of the pulse oximeters studied,32 but shielding of the probe from ambient light is often used clinically to improve performance. Hypoperfusion also may limit the ability of pulse oximeters to adequately detect a pulsatile signal and can adversely affect reported saturation values.31
The presence of interfering dyes or dyshemoglobinopathies is an infrequent clinical problem but can result in erroneous pulse oximetry readings. In methemoglobinemia, the iron in the heme groups in hemoglobin becomes oxidized from the ferrous (Fe2+) state to the ferric (Fe3+) state. The oxidized form of hemoglobin, called methemoglobin, cannot bind oxygen. Thus the presence of significant quantities of methemoglobin leads to tissue hypoxia because these molecules no longer participate in oxygen transport. However, light absorbance by methemoglobin more closely resembles oxyhemoglobin than deoxyhemoglobin at the measured wavelengths, erroneously leading the pulse oximeter to indicate a higher percentage of oxygen saturation than expected.33,34 Similarly, the presence of carboxyhemoglobin may result in erroneous reading in pulse oximetry because carbon monoxide–bound hemoglobin also does not participate in oxygen transport.35
Masimo has recently introduced a pulse oximeter, known as the Masimo Rainbow SET, that uses eight wavelengths of light (Figure 43-3) and thus is able to determine carboxyhemoglobin and methemoglobin levels in addition to oxygenated and deoxygenated hemoglobin.36 Because these other species of hemoglobin are recognized, it is also possible to have a continuous readout of total hemoglobin. The Rainbow oximeter will be useful not only for monitoring patients with dyshemoglobinemias but also in situations where occult blood loss may be occurring because of its ability to report total hemoglobin. Because the technology is relatively new, confirmation with laboratory samples may still be needed, but trends can then be followed with the oximeter, minimizing the need for blood sampling.37
Because cooximeters account for the presence of both carboxyhemoglobin and methemoglobin, blood gas samples sent for cooximetry should correctly measure hemoglobin saturation in cases where measurable levels of either methemoglobin or carboxyhemoglobin are present or suspected. Fetal hemoglobin has a sufficiently similar absorbance spectrum to adult hemoglobin, such that the presence of fetal hemoglobin does not significantly affect the determined saturation.38 The presence of bilirubin also does not appear to significantly affect pulse oximetry readings.39
Probe Placement
Pulse oximetry probes typically are placed on fingers or toes, with the light-emitting diodes placed across the digit, opposite from the detector. For premature and small infants, the probe often is placed around the entire palm or foot with good results. Because of scattering of light in tissue, pulse oximeter probes also can be used in a reflectance mode. In this manner, both light-emitting diodes and detectors are on the same surface and can be placed, for example, on the forehead.40,41 Transesophageal probes have been designed and are used for care of operative or critically ill patients with potentially poor peripheral perfusion.42–44
Cerebral Oximetry
The basic principles underlying pulse oximetry have been extended to determine hemoglobin saturation in the brain. Currently a small number of devices for determination of cerebral oxygenation are commercially available. Somanetics and Casmed both have FDA-approved devices available for pediatric use. Hamamatsu also makes a device, the NIRO 300, but it currently has not been approved by the FDA.45 These devices take advantage of the relative transparency of the skull and brain in the near-infrared spectral region. However, because these devices do not restrict analyses to a pulsatile component, the information provided comes from light absorption of hemoglobin in arterial, venous, and capillary blood and is contaminated to some extent by the presence of other light-absorbing molecules, primarily the cytochromes. Thus these devices provide a “relative” saturation value of cerebral oxygenation, which may have some correlation with clinical conditions,46 but these devices currently do not report an absolute saturation value in the way that pulse oximeters do. Technical advances likely will improve the clinical utility of cerebral oximetry as the information provided becomes more reliable.
Somatic Regional Oximetry
Near infra-red spectroscopy (NIRS) also has been used to measure somatic regional oxygen saturation (rSO2). In one resusucitation study of children with moderate dehydration, NIRS probes were placed on the forehead to reflect cerebral rSO2 and on the flank to reflect somatic rSO2.. In children with moderate dehydration the cerebral rSO2 was preserved but the somatic rSO2 demonstrated regional hypoperfusion when the patients were dehydrated and showed an increase with rehydration.47 Similarly, NIRS was used to provide cerebral and somatic oxyhemoglobin data for a group of neonates with hypoplastic left heart who were awaiting palliation, and it was found to simplify management, allowing more accurate assessment of systemic perfusion.48
Muscle Oximetry
Optical spectroscopy has been used to assess muscle oxygenation with increasing success. Earlier approaches for determining muscle oxygenation have been limited by the similarity between optical absorbance spectra from hemoglobin and myoglobin. Many reports of tissue oxygenation as a combined hemoglobin plus myoglobin saturation have been reported.49 Because hemoglobin and myoglobin have vastly different oxygen dissociation relationships,50 a combined saturation may have little clinical significance. Successful distinction of myoglobin saturation from hemoglobin saturation has been reported, using a complex multiwavelength spectra analytic approach.51,52 Because myoglobin is an intracellular oxygen-binding molecule in cardiac and skeletal muscle, myoglobin saturation can be used to determine intracellular oxygen tension. This approach has led to the determination of intracellular oxygen tension measurements in both cardiac and skeletal muscle in laboratory studies.53–55 Technical advances using this approach likely will have a significant impact on clinical monitoring of critically ill and injured patients.
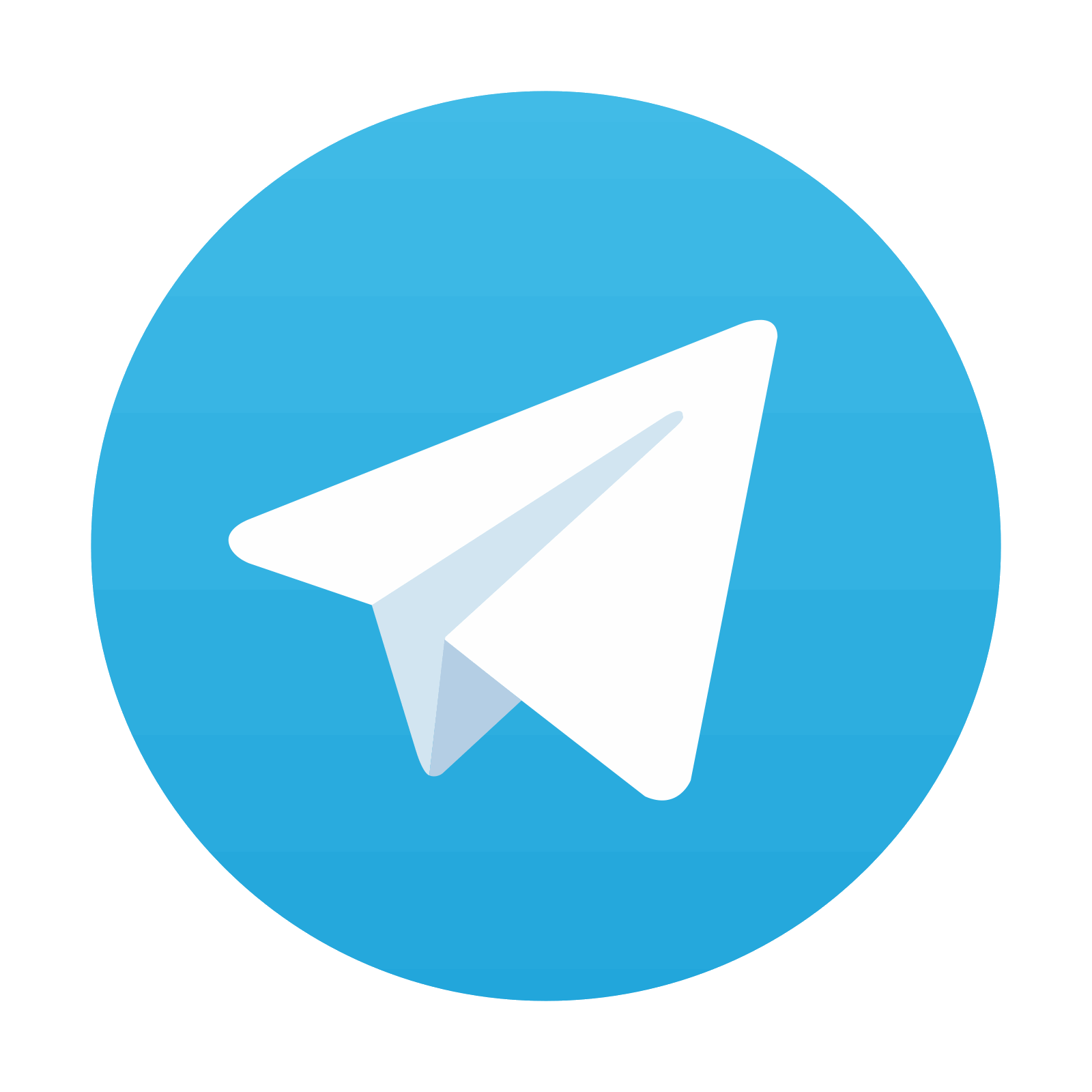
Stay updated, free articles. Join our Telegram channel
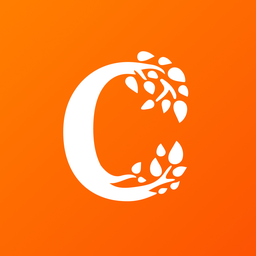
Full access? Get Clinical Tree
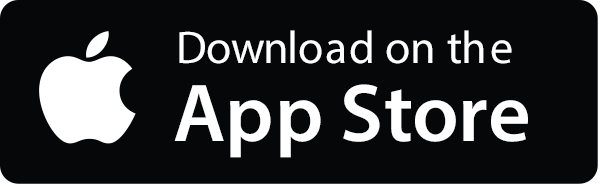
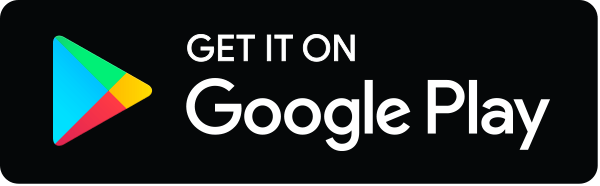