Noninvasive Hemodynamic Monitoring
BACKGROUND
The provision of optimal medical care presents a dilemma in emergency medicine. As the importance of early resuscitation continues to be reinforced, the role and responsibilities of the emergency physician continue to expand.1,2 Unfortunately, overcrowded emergency departments and overextended staff weaken the emergency physician’s ability to provide the highest level of care to the sickest patients.3–5 This concerning trend is exemplified in the early management of severe sepsis, in which full realization of the benefits of aggressive goal-directed therapy is often constrained by the requirement for invasive monitoring.6,7 Knowledge of noninvasive hemodynamic monitoring modalities enables the emergency physician to improve diagnostic efficiency and more effectively deliver care to the critically ill.8 This chapter reviews the noninvasive monitoring devices available to the emergency physician and discusses their clinical applicability.
CARDIAC OUTPUT MONITORING
As discussed in detail in Chapter 2, tissue oxygenation is maintained during periods of rising metabolic demand by the modulation of cardiac output and tissue oxygen extraction. If cardiac output or arterial oxygen content is suboptimal, the delivery of oxygen, or tissue perfusion, may drop below a critical threshold, and the body’s oxygen consumption becomes supply dependent. If this supply-dependent phase is not rapidly corrected, tissues enter a dysoxic state and a shock ensues (Fig. 3.1).
FIGURE 3.1 The relationship between oxygen delivery (DO2), oxygen consumption (VO2), and oxygen extraction ratio (OER). As DO2 decreases, tissue OER increases in order to maintain constant VO2. However, beyond a certain critical DO2 at which OER is maximized, VO2 becomes supply dependent, oxygen deficit accumulates, and a shock state ensues. Figure courtesy of Chad M. Meyers, MD.
The ability of physicians to make reliable clinical estimates of cardiac output is limited. Emergency physicians, intensivists, and surgeons were consistently unable to provide accurate clinical assessment of hemodynamics when compared to invasive and noninvasive determination of cardiac output and systemic vascular resistance.9–13 This limitation likely reflects an overdependence on measurements such as blood pressure and heart rate, neither of which are reliable indicators of cardiac output or critical illness.14,15 A significant number of critically ill patients will present with normal vital signs despite having global tissue hypoxia, identified by an elevated lactate or abnormal central venous oxygen saturation (Scv02).16 Noninvasive cardiac monitors enable the emergency physician to identify concerning trends in cardiac function present in critically ill patients prior to the development of significant hemodynamic instability; importantly, this information not only helps guide management but also has been shown to predict outcome.17–19
Management of compromised cardiac output requires a basic understanding of the determinants of cardiac function. Cardiac output is the product of stroke volume and heart rate. Stroke volume, in turn, is dependent on preload, afterload, and the quality of cardiac contractility.20 Each of these variables may be estimated and manipulated to optimize forward flow. For example, an echocardiogram with evidence of poor contractility may prompt the administration of inotropic agents. The most common initial therapeutic strategy, however, is restoration of homeostasis, which typically begins with an assessment of preload and an attempt to determine fluid responsiveness.
IMPORTANCE OF FLUID RESPONSIVENESS
The importance of fluid resuscitation in the management of the critically ill patient cannot be overstated. Fluid resuscitation using objective endpoints has been found to improve clinical outcomes in various clinical settings, while overzealous resuscitation has been found to increase mortality.7,21–30 Ultimately, the decision to administer fluid to a patient in shock is driven by the goal of improving cardiac output as a means of restoring adequate tissue perfusion. This concept is referred to as fluid responsiveness and implies residence on the ascending portion of the Frank-Starling curve. Historically, the determination of fluid responsiveness relied on static estimates of preload such as central venous pressure (CVP) and pulmonary artery occlusion pressure (PAOP). However, as discussed in Chapter 2, static pressure–based measurements have repeatedly been shown to be poor predictors of fluid responsiveness.31–36 While the adequacy of preload largely determines response to fluid administration, the interplay between venous return and cardiac output is also dependent on the contractile state of the heart and afterload, both of which change unpredictably in patients with critical illness. Thus, an increase in right-sided filling pressure that would normally improve cardiac output may correspond to the flat, nonrecruitable portion of the cardiac function curve in the same patient with critical illness associated as pathologic alterations in cardiac contractility (Fig. 3.2).37–40 In this circumstance, further fluid resuscitation would do little to improve cardiac output and risk fluid overload.
FIGURE 3.2 In Guyton’s depiction of cardiocirculatory function, the intersection of the two curves represents the current cardiac output and right atrial pressure for any given combination of venous return and cardiac function states. In the patient with normal cardiac function who is operating on the ascending fluid-responsive portion of the Frank-Starling curve, the infusion of volume results in a rightward shift of the venous return curve, with subsequent increase in venous return, preload, right atrial pressure, and, ultimately, cardiac output. In contrast, in the patient with decreased cardiac performance whose venous return/cardiac function intersection already lies on the flat unresponsive portion of the Frank-Starling curve, further infusion of volume will not result in increased cardiac output, instead only resulting in increased resistive right atrial filling pressures and likely volume overload. Figure courtesy of Chad M. Meyers, MD.
In contrast to static measurements of preload like CVP that provide limited information regarding the potential for fluids to enhance forward flow, noninvasive monitors attempt to measure, either directly or indirectly, dynamic changes in cardiac output as a function of filling pressure. The calculations used to generate these indices are predicated on heart–lung interactions in which venous return, cardiac filling pressure, and cardiac output change as a result of increasing and decreasing intrathoracic pressure during the respiratory cycle. The reproducibility of these indices, however, is currently limited to sedated and mechanically ventilated patients in whom tidal volumes, and therefore changes in intrathoracic pressure, remain relatively constant over time. While useful indices of fluid responsiveness in spontaneously breathing patients do exist, nearly all studies demonstrating reliable prediction of fluid responsiveness have done so in ventilated patients without spontaneous breathing and with tidal volumes of >8 mL/kg.
THE SCIENCE OF DEVICE ASSESSMENT
The evaluation of a novel noninvasive cardiac output monitor requires assessment of both the reliability of its measurements and the validity of its clinical application. Since the inception of the pulmonary artery catheter (PAC), thermodilution-based measurements of cardiac output are the standard to which new devices are compared and reliability assessed. Early studies comparing cardiac monitors used linear regression and correlation in their analysis. The error of such methods is that they focused on the relationship between measurements and not their agreement; that is, two monitors might correlate well but produce substantially different measurements. Bland and Altman realized the limitation of such methodology and introduced the concepts of bias and precision when comparing a new method of clinical measurement to a standard reference.41,42 This type of analysis provides more useful information regarding the interchangeability of two measurement devices.
What is considered an acceptable limit of agreement, however, is controversial.43–45 A 1999 meta-analysis of noninvasive cardiac output monitors proposed that a percentage error of ± 30% be adopted as the acceptable limit of error between a novel cardiac monitor and bolus thermodilution.44 The reliability of thermodilution, however, is itself disputed.46 One intraoperative study using magnetic aortic flowmetry—the original gold standard for cardiac output—noted the percent error of PAC thermodilution for measuring cardiac output to be as high as 46%.47 Ultimately, very few cardiac monitors have consistently achieved the 30% threshold for acceptable reliability, arguably because of the limitations of PAC thermodilution to which they are being compared. To accommodate this variability, it was proposed that the standard for acceptable level of agreement for a novel cardiac output monitor be increased to ± 45%.45 The implications of such a wide range of agreement are, however, considerable, and discussion regarding what constitutes acceptable reliability continues. The current goal for a novel cardiac output monitor remains 30% agreement with PAC bolus thermodilution.48
While device reliability is important, the validity of its clinical application is arguably of greater significance. It is now recognized that the most important indicators of adequate resuscitation are not specific quantitative measures, per se, but rather the qualitative adequacy of cardiac output. To this end, assessment of monitor performance is increasingly linked to the ability to predict physiologic determinants of cardiac output such as euvolemia and volume responsiveness. Analysis of this performance is often described in terms of a receiver operating characteristic (ROC) curve. The “area under the curve,” or AUC, represents the relationship between true and false positives over a range of thresholds of positivity and provides an overall assessment of device performance. AUC values range between 0.5 (a random association between test result and outcome) and 1, a perfect prediction. In addition, the slope of a line connecting any two points along the ROC represents the likelihood ratio for that specific interval, allowing the clinician to avoid the limitations of single dichotomous yes/no clinical cutoffs and improving context-specific applicability.49–51
PRINCIPALS OF MINIMALLY INVASIVE ASSESSMENT OF FLUID RESPONSIVENESS
Arterial Waveform Analysis
Various dynamic methods of arterial waveform analysis, both minimally invasive and completely noninvasive, have been identified to assist in the determination of fluid responsiveness in the mechanically ventilated patient. As venous return decreases with positive pressure ventilation, a patient functioning on the ascending portion of the Frank-Starling curve will demonstrate a transient corresponding decrease in cardiac output. If arterial pressure is continuously transduced in the presence of sinus rhythm, this decrease in cardiac output manifests as a change in the arterial waveform several beats later.52 Several calculations capturing this relationship are described in the following sections.
Delta-down
Delta-down (d-down) is defined as the difference, in mm Hg, between the minimum systolic pressure over several respiratory cycles and a reference systolic pressure measured during an end-expiratory pause. One study demonstrated that a d-down >5 mm Hg had a positive predictive value of 95% and a negative predictive value of 93% for fluid responders and nonresponders, respectively. The magnitude of the d-down correlated linearly with the corresponding increase in cardiac output achieved with additional fluids.53
Pulse Pressure Variation
Pulse pressure variation (PPV) is defined as the difference between the maximal and minimal pulse pressures that occurs during the respiratory cycle (Fig. 3.3). Various diagnostic PPV thresholds are described, with two studies demonstrating values of >13% or >11% to have a positive predictive value of 94% or 100% and a negative predictive value of 96% and 93%, respectively.54,55 In a meta-analysis comparing intravascular arterial modalities, PPV had the greatest diagnostic accuracy for fluid-responsive states, when compared to other such measures including systolic pressure variation (SPV) and pulse contour estimates of stroke volume variation (SVV), to be discussed below.53
FIGURE 3.3 Various methods of arterial waveform analysis can assist with the determination of fluid responsiveness in mechanically ventilated patients without spontaneous breathing. PPV is the difference between maximal (PPmax) and minimal (PPmin) pulse pressure over the respiratory cycle. Delta-down (∆Down) is defined as the difference between the systolic pressure of a reference pulse pressure (PPref) measured during an expiratory hold and the minimal systolic pressure during the respiratory cycle. SPV is defined as the total change in systolic pressure over the respiratory cycle and is the sum of delta-down and delta-up (∆Up). Figure courtesy of Chad M. Meyers, MD.
Pulse Oximeter Waveform Analysis
The photoplethysmogram, or waveform, displayed by transmission pulse oximeters represents the variation in light intensity transmitted through the fingertip or ear lobe. The intensity of this transmitted light is inversely related to intravascular blood volume. Similar in appearance to arterial blood pressure waveforms, the pulse oximeter waveform is traditionally used by the clinician to distinguish between physiologic and noisy waveforms, in order to determine the reliability of arterial oxygen saturation measurements.56
Photoplethysmographic waveforms also permit the calculation of several completely noninvasive indices that have been shown to correlate with fluid responsiveness in ventilated patients without spontaneous respirations. A respiratory variation in pulse oximetry plethysmographic waveform amplitude, or d-POP, of >13% was found to predict fluid responsiveness with 80% sensitivity and 90% specificity when compared to its intravascular physiologic correlate PPV.57 Another photoplethysmographic measure, the perfusion index (PI), describes the relationship between the pulsatile and nonpulsatile plethysmographic signal. Changes in PI are monitored over the respiratory cycle using a specialized pulse oximeter (Masimo Corp, Irvine, CA) and are displayed as the plethysmographic variability index (PVI), allowing for continuous assessment of fluid responsiveness. In a series of 25 patients after induction of general anesthesia, a PVI >14% was found to discriminate responders with 81% sensitivity and 100% specificity.58
A meta-analysis of 10 studies investigating d-POP and PVI found inferior correlation with each other when predicting the magnitude of corresponding cardiac output change, but suggested similar predictive value in the determination of fluid responsiveness when compared to PPV.59 However, while such simple noninvasive determinants of fluid responsiveness are appealing, the accuracy of d-POP and PVI are dependent on the quality of the plethysmographic waveform. Two studies observing ICU patients receiving norepinephrine infusions with corresponding vasoactive drug-induced changes in peripheral vascular tone found significantly reduced correlation between both d-POP/PVI and PPV, suggesting limited applicability in the critically ill hemodynamically unstable patient.60,61
STROKE VOLUME VARIATION
Intermittent determination of cardiac output by methods such as bolus thermodilution provide the clinician with broad trends in cardiac function, but are limited in periods of rapid hemodynamic change common in critical illness. Cardiac output monitors capable of continuous beat-to-beat stroke volume assessment not only assist the emergency physician with earlier recognition of sudden changes in cardiovascular function but also provide a unique dynamic marker of fluid responsiveness. A meta-analysis of 23 studies investigating the relationship between fluid responsiveness and SVV as determined by a variety of devices (PiCCO, LiDCO, FloTrac, and USCOM) found a pooled sensitivity and specificity of 81% and 80%, respectively, and an area under the receiver operating characteristic curve (AUC) of 0.84.62 A similar meta-analysis supported these findings, but demonstrated slightly weaker predictive values when comparing SVV to PPV and SPV, the AUC of which were 0.94 and 0.86, respectively.53 While most of these studies were performed in the operating room under general anesthesia, eight were performed in the ICU with heterogeneous mechanically ventilated patient populations without spontaneous breathing. In another study that investigated intubated septic shock patients spontaneously breathing on the mechanical ventilator, the AUC was reduced to 0.52, prompting investigators to conclude that SVV is not of value in spontaneously breathing patients.63 Additionally, nearly all studies demonstrating the utility of arterial waveform measures (PPV, SPV, SVV) in ventilated patients did so in patients receiving tidal volumes of >8 mL/kg.53,62 In studies in which patients received tidal volumes of <8 mL/kg (typically acute respiratory distress syndrome [ARDS] patients following low TV protocols), this relationship was not maintained.64,65
ULTRASOUND INDICES
Inferior Vena Cava
Echocardiographic evaluation of the inferior vena cava (IVC) using the subcostal view provides the clinician with a noninvasive estimation of right heart filling pressure and fluid responsiveness in mechanically ventilated patients. As intrathoracic pressure increases and decreases with positive pressure ventilation, low venous return and low right-sided filling pressures (i.e., low intravascular volume) result in an increase in both right atrial and IVC compliance, which manifests as respirophasic change in the diameter of the IVC.38 A study measuring cardiac output in septic patients before and after volume expansion with colloids demonstrated that a distensibility index of the IVC (dIVC) of >18% has a sensitivity of 90% and specificity of 90% for the determination of fluid responsiveness (Table 3.1). A second study, also evaluating fluid responsiveness, demonstrated a dIVC of >12% to have a positive predictive value of 93% and negative predictive value of 92%.66,67 Of note, the application of IVC ultrasound may be limited in obese or postoperative laparotomy patients.
TABLE 3.1 Formulas and Thresholds for the Determination of Fluid Responsiveness
Brachial Artery Peak Velocity Variation
Similar to arterial waveform analysis, noninvasive Doppler assessment of brachial artery flow over the respiratory cycle can identify volume-responsive patients. This technique requires the patient be mechanically ventilated without spontaneous breathing and in sinus rhythm. When brachial artery maximum and minimum velocities were recorded during standardized ventilation with a handheld ultrasound device, a brachial artery peak velocity variation (d-Vpeakbrach) >16% was found to correlate with PPV.68 In a separate case series, d-Vpeakbrach > 10% was found to predict fluid responsiveness with a sensitivity of 74% and specificity of 95%.69
MEASUREMENTS OF VOLUME RESPONSIVENESS IN SPONTANEOUSLY BREATHING PATIENTS
The reliance of dynamic indices on positive changes in intrathoracic pressure limits their applicability in spontaneously breathing patients. While a single study found that a drop in right atrial pressure >1 mm Hg (as measured by CVP) during inspiration predicted volume response in spontaneously breathing patients, most other studies have not duplicated these findings.31 One study found that SVV in mechanically ventilated patients who were breathing spontaneously with pressure support did not have value in predicting fluid responsiveness, with an AUC of 0.52.63 In spontaneously breathing patients who are significantly volume depleted, these indices may still be of some value. In a study of hemodynamically unstable and clinically volume-depleted patients, PPV >12% was found to be 92% specific for identifying volume responsiveness, but the absence of PPV had poor sensitivity (63%) for excluding hypovolemia.70 Significant IVC variation may also have value in identification of fluid responsiveness. In a study of 40 hemodynamically unstable patients, IVC collapse of >40% had a sensitivity and specificity of 70% and 80%, respectively, for fluid responders.71
Passive Leg Raise
The simplest provocative test of fluid responsiveness is to administer a fluid bolus and then measure whether an appropriate increase in cardiac output has occurred. This fluid challenge can be extrinsic, in the form of a bolus of crystalloid or colloid fluids, or intrinsic, in the form of autotransfused lower extremity blood volume achieved by passive leg raise (PLR). PLR is performed by elevating the lower extremities 30 to 45 degrees relative to the upper body in supine position for a period of 1 to 5 minutes and then measuring cardiac output invasively or noninvasively. An increase in cardiac output of 10% to 15% after PLR predicts fluid responsiveness.72–81
The PLR-induced change in cardiac output (PLR-cCO) technique is capable of accurately determining fluid responsiveness in mechanically ventilated or spontaneously breathing patients and is not affected by the presence of cardiac dysrhythmias. In one meta-analysis, which reviewed the applicability of PLR-cCO in the ICU—and included patients spontaneously breathing or mechanically ventilated—the pooled sensitivity and specificity for fluid-responsive patients were 89.4% and 91.4%, respectively, with an AUC of 0.95.77
PLR-induced changes in arterial pulse pressure (PLR-cPP) have also been evaluated for their ability to determine fluid responsiveness in spontaneously breathing patients with normal sinus rhythm. In a case series of 34 nonintubated patients, PLR-induced changes in radial pulse pressure >9% were shown to predict fluid responsiveness with a sensitivity of 79% and specificity of 85%.76 However, in a meta-analysis, including both spontaneously breathing and mechanically ventilated patients, PLR-cPP demonstrated significantly lower predictive value for fluid responsiveness than PLR-cCO, with a pooled sensitivity and specificity of 59.5% and 86.2%, respectively, and an AUC of 0.76.77
End-Tidal CO2
In mechanically ventilated patients with stable minute ventilation and stable tissue carbon dioxide (CO2) production, continuous capnometry may provide a simple method to determine fluid response. Two studies demonstrated that an end-tidal CO2 (EtCO2) increases >5% in response to PLR-predicted fluid response with a sensitivity of 71% and 90.5% and a specificity of 100% and 93.7%, respectively.82,83
SPECIFIC DEVICES AND TECHNIQUES FOR THE ASSESSMENT OF CARDIAC OUTPUT
Pulse Contour Analysis
Several devices are available that indirectly estimate cardiac output by changes in the contour of the arterial waveform over the cardiac cycle. Derived from Otto Frank’s Windkessel model published in 1899, pulse contour analysis estimates beat-to-beat cardiac output based on the relationship between blood pressure, stroke volume, arterial compliance, and vascular resistance.84,85 While each device uses a different method to calculate the variables in the Windkessel relationship, they can be broadly divided into two main categories: calibrated and uncalibrated.86
The two calibrated devices, the Pulse Contour Cardiac Output, or PiCCO (Pulsion Medical Systems SE, Munich, Germany), and Lithium Detected Cardiac Output, or LiDCO (LiDCO Ltd, London, UK), compensate for individual differences in vascular impedance by intermittent direct determination of cardiac output using indicator dilution techniques. Beat-to-beat cardiac output is then calculated using continuous measurement of the area under the systolic portion of the arterial pulse wave.87 PiCCO uses transpulmonary thermodilution to determine cardiac output, a method that requires both central venous and central arterial cannulation.88 The LiDCO monitor uses lithium bolus dilution and uses an arterial catheter paired with either a peripheral or central venous catheter.89,90 Both devices require recalibration via indicator dilution every 4 to 6 hours or whenever a change in patient status occurs.91,92
Uncalibrated devices determine cardiac output by mathematically estimating aortic impedance and vascular resistance. Beat-to-beat cardiac output is then calculated in a similar manner as calibrated devices. The most widely tested of the uncalibrated devices, the FloTrac (Edwards Lifesciences, Irvine, CA), requires only peripheral artery catheterization and standard patient characteristics to estimate large-vessel compliance and estimate cardiac output.93,94 An additional uncalibrated device, Nexfin (Edwards Lifesciences, Irvine, CA), uses the volume-clamp principle to estimate arterial pressure via an inflatable finger cuff, calculating cardiac output via an updated Modelflow algorithm that allows for a completely noninvasive monitoring modality.95,96
When compared to PAC thermodilution, the reliability of pulse contour analysis for the estimation of cardiac output varies greatly. PiCCO device estimates of cardiac output have been found to correlate acceptably with thermodilution; however, these studies have shown conflicting results during periods of hemodynamic instability.91,97,98 One study demonstrated that PiCCO remained reliable when compared to transpulmonary thermodilution in septic patients with circulatory failure.99 However, two additional studies demonstrated a percentage error of >30% if the device was not recalibrated hourly during periods of hemodynamic instability or following therapeutic maneuvers such as fluid challenge.100,101
The LiDCO system has been found to correlate well with thermodilution both intra- and postoperatively, but has not been well studied during periods of hemodynamic instability.89,90,102 The FloTrac device has been studied widely given its simplicity, minimal invasiveness, and ease of use; however, results have been inconsistent and mostly disappointing.86 Despite several generations of software and hardware updates, the FloTrac has generally shown an unacceptable cardiac output agreement with PAC thermodilution.45,86,103–108 Although a meta-analysis of 16 prospective investigations found an improvement in the accuracy and precision of the FloTrac when comparing earlier versions of the device and more recent versions, the review was criticized for excluding studies that evaluated hemodynamically unstable patients.45,109 A subgroup analysis of the newer generation of FloTrac devices found a percentage error of 44.7% when compared to PAC thermodilution when septic and critically ill patient populations were included.45
A meta-analysis of 24 studies in mixed patient populations comparing PiCCO, LiDCO, and FloTrac with PAC thermodilution found a pooled percentage error of 41.3% for all three pulse contour monitors.45 In another comparative study of LiDCO, PiCCO, and FloTrac, LiDCO was found to have the best overall agreement when compared to PAC thermodilution, with a percentage agreement of 29%, compared to 41% and 59% for PiCCO and FloTrac, respectively.106,110 The Nexfin has not been extensively studied, but preliminary results are promising when compared to thermodilution intraoperatively and in the cardiac ICU.111–113
Ultimately, while the simplicity of uncalibrated devices such as FloTrac makes application in the ED attractive, the poor performance of the current models limits their applicability. The calibrated PiCCO and LiDCO devices demonstrate improved reliability when compared to thermodilution, but are limited by the need for frequent recalibration during periods of hemodynamic instability or following therapeutic intervention. In addition, the PiCCO device requires central venous and central arterial cannulation for calibration by transpulmonary thermodilution, which limits its applicability.
Ultrasound
Esophageal Doppler
Esophageal Doppler allows continuous estimation of stroke volume by recording the velocity of blood flow in the descending aorta with a flexible ultrasound probe—roughly the size of a nasogastric tube—placed either nasally or orally in a mechanically ventilated patient. The accurate assessment of stroke volume via esophageal measurement relies on two assumptions: (1) the presence of a stable cephalic-to-caudal blood flow ratio, which may be inconsistent in certain patients, and (2) a homogenous cross-sectional area of the aorta. Aortic measurement is estimated either by calculations based on height, weight, and age or directly via real-time motion-mode (M-mode) Doppler on capable models.114 While esophageal Doppler is highly operator dependent, it is quickly performed, and one study suggests no more than 12 placements are needed to become proficient.114 In a prospective study in the emergency department (ED), mean time until optimal Doppler signal was obtained was 5.7 minutes.115
The reliability of esophageal Doppler to estimate cardiac output when compared to PAC thermodilution is, however, not encouraging. Two separate meta-analyses demonstrated limits of agreement of ± 65% and 42.1%, respectively.44,45 A meta-analysis of 11 validation studies also found limited agreement in cardiac output values when compared to PAC thermodilution, but improved results when following trends. The limited number of studies included in the review does make it difficult to draw conclusions regarding the accuracy of these findings.116 Analyzed separately, studies comparing M-mode–capable esophageal Doppler with PAC thermodilution have revealed better agreement and may provide the clinician with a valuable method to continuously measure cardiac output in the mechanically ventilated patient.117–119
USCOM Cardiac Monitor
The ultrasonic cardiac output monitor, or USCOM (USCOM LTD, Sydney, Australia), is a completely noninvasive cardiac output monitoring system that uses transthoracic Doppler to measure either transaortic or transpulmonary blood flow. Stroke volume is calculated by measurement of the velocity time integral (VTI) and the cross-sectional area of the outflow tract.86,120 Clinical trials comparing USCOM with thermodilution have shown conflicting results.121–124 In one meta-analysis, the range of percentage error in the 10 studies reviewed was between 14% and 56%, with a pooled percentage error of 42.7%.120 In addition, like esophageal Doppler, the USCOM device is user dependent, with a failure rate to obtain measurements ranging from 5% to 24% across studies.120,122,124–126
Transthoracic Echocardiography
Transthoracic echocardiography (TTE) provides the emergency physician with indispensable clinical information regarding left and right cardiac function, ventricular dilation, pericardial effusion, and valvular competence.86,127,128 As with the USCOM device, TTE can provide intermittent quantitative estimation of cardiac output using Doppler assessment of the left ventricular outflow tract (LVOT) and VTI calculations. Basic qualitative information regarding adequacy of cardiac filling and global function is also easily obtained. While providing no numerical data regarding cardiac output, a brief and focused qualitative exam can often identify patients that need more fluid versus those that are euvolemic and distinguish between patients needing inotropes from those with normal function. A detailed discussion of echocardiographic evaluation of the critically ill patients is presented in Chapter 6.
While the utility of transthoracic LVOT assessment to follow cardiac output changes is limited given the necessity of repeat measurements and time-consuming nature of the study, it may have a role in the absence of other specialized devices.
Transthoracic Electrical Bioimpedance
Transthoracic electrical bioimpedance (TEB) estimates cardiac output by measuring resistance in the thorax to high-frequency, low-magnitude current to determine changes in total thoracic fluid content over the cardiac cycle. First introduced in the 1970s, cardiac bioimpedance monitors use skin electrodes applied to the neck and thorax, allowing for quick and completely noninvasive cardiac monitoring.129,130 TEB is limited in its ability to assess cardiac output in patients with dysrhythmias or pulmonary edema.87,129,131 In two studies evaluating critically ill ED patients, estimation of cardiac output using TEB agreed well with PAC thermodilution, but accuracy was compromised in the presence of pulmonary edema, pleural effusions, chest wall edema, or chest tubes.18,132 However, when clinical studies comparing TEB with PAC thermodilution were systematically reviewed, the findings were less favorable. A 1999 meta-analysis estimated the percentage error of TEB compared with PAC thermodilution to be ± 38%.44 A more recent meta-analysis echoed the limitation of TEB, estimating an overall mean percent error of ± 42.9%.45 A newer noninvasive cardiac output monitor, or NICOM device (NICOM, Cheetah Medical Inc., Wilmington, DE), using the related principle of bioreactance, has been less rigorously studied. It is unclear at this time whether it shares the same limitations as TEB.133–136
Carbon Dioxide and the Fick Principle
The Fick Equation, which is based on the conservation of mass, allows for the calculation of cardiac output (or alveolar blood flow) by measuring the arteriovenous difference in oxygen consumption. The Fick principle may be applied to any gas diffusing through the lungs, including carbon dioxide.8 These concepts are discussed in detail in Chapter 2.
The noninvasive cardiac output device, or NICO (Novametrix Medical Systems Inc., Wallingford, CT), uses a partial carbon dioxide rebreathing technique in intubated patients to estimate CO2 elimination (VCO2) and uses the changes in venous and arterial CO2 EtCO2 to calculate the aretiorvenous difference in CO2. However, to accurately assess cardiac output by expired CO2, pulmonary capillary blood flow must also be known. To determine this, pulmonary shunting is estimated using SpO2 and FiO2; thus, the NICO provides a noninvasive determination of cardiac output using mainstream capnometry, a differential pressure pneumotachometer, and pulse oximetry.137 One shortcoming of this technique is that EtCO2 provides unreliable estimates of PaCO2 in unstable patients due to the variability of V/Q mismatch in the critically ill. Estimating pulmonary capillary blood flow can compensate for this, but several studies have demonstrated the calculated pulmonary shunt fraction by NICO differs considerably from traditionally calculated shunt fraction by blood gas analysis.138,139 In light of this, cardiac output calculated by NICO was typically only considered accurate when limited to stable patients with normal pulmonary function or when monitoring trends.87 This was supported by a pooled analysis of 167 measurements from 8 studies performed in various patient populations, which found a mean percentage error of ± 44.5% for partial CO2 rebreathing method.45 At this time, the NICO may be more appropriate for anesthetized patients in the operating room rather than in the stabilization of critically ill patients in the ED.
A PRACTICAL FLUID STRATEGY IN SEVERE SEPSIS
Despite evidence of significant reduction in mortality when used to guide therapy in patients with severe sepsis and septic shock, the adoption of early goal-directed therapy (EGDT) is not widespread.6,140–142 One barrier to implementation is the requirement for central venous access and specialized monitoring equipment.6,140 In hemodynamically stable patients who meet the criteria for severe sepsis by elevated lactate alone, and who do not require central access for vasopressor support, adherence to the EGDT algorithm’s requirement for central access is understandably reduced.143,144 Central venous catheterization is not a benign procedure, and the clinical information gained by knowledge of the CVP and central venous O2 (ScvO2) saturation must be balanced with the inherent risk of invasive monitoring.145–147 Furthermore, in a recent landmark study, lactate clearance compared favorably with ScvO2 as a final resuscitative endpoint in patients with severe sepsis.148
Aggressive fluid therapy, however, remains a cornerstone of management in sepsis, and despite the well-documented limitations of CVP in predicting fluid responsiveness, its use has been found to significantly increase the volume of fluid administered in the first 6 hours of therapy.7 The additional fluids given in the EGDT trial’s experimental group are thought to have played a central role in the observed positive impact on mortality. In the trial, the control group resuscitated empirically received on average 3.5 ± 2.4 L crystalloid, compared with the experimental group guided by a target CVP of 8 to 12 mm Hg, which received 4.9 ± 2.9 L.7 Other studies have found a similar disparity between empiric and targeted volume resuscitation in severe sepsis.143 Importantly, despite the significantly larger volume of fluid given, respiratory failure requiring mechanical ventilation was not more frequent in the EGDT group. Therefore, while CVP does not adequately predict fluid responsiveness, it may demonstrate the concept of fluid tolerance: A patient with severe sepsis who is not volume overloaded will likely benefit from additional fluid therapy.
Using measures of fluid responsiveness to guide therapy in sepsis is ideal. However, indices of fluid responsiveness can be cumbersome, time consuming, and often require the patient to be mechanically ventilated without spontaneous respirations. In contrast, fluid tolerance can be quickly assessed with an ultrasound survey of the IVC, heart, and lungs and is a valuable initial assessment tool. While IVC variation has limited capability to predict fluid responsiveness in spontaneously breathing patients, a collapse of >50% has been demonstrated to correlate well with CVP <8 mm Hg.149,150 In patients in whom adequate visualization of the IVC is difficult, ultrasound of the bilateral apical three interspaces provides an alternative approach to estimating cardiac filling pressures. One study—while investigating fluid tolerance in the ICU—demonstrated that <3 b-lines (a comet tail artifact indicating subpleural interstitial edema) per interspace identified a pulmonary artery opening pressure, or PAOP, of <18 mm Hg with a PPV of 97%.151 It should be noted, while the absence of lung ultrasound b-lines is indicative of low filling pressures, their presence is too sensitive and does not accurately reflect high filling pressures.151 Therefore, while the development of b-lines should prompt caution, it may not accurately reflect the transition past the upper inflection point of the Frank-Starling curve.
Indicators of fluid tolerance are not meant to replace those of fluid responsiveness. Instead, they are meant to supplement the preliminary clinical evaluation and increase the clinician’s confidence in initial volume resuscitation. If, during the administration of fluid, the patient develops clinical evidence of volume overload—or indices of fluid tolerance are lost and a subsequent repeat serum lactate has not normalized—then more time-intensive measures of fluid responsiveness are justified to guide fluid therapy.
Finally, qualitative assessment of ejection fraction with early standardized echocardiography is always recommended as it provides the clinician with an overall estimate of cardiac function and has been demonstrated to improve survival and ventilator-free days when performed in the ICU.152
CONCLUSION
While knowledge of hemodynamics plays an important role in the ED resuscitation of the critically ill, the determination of cardiac function has historically been limited to the intensivist. With the advent of noninvasive hemodynamic monitoring, emergency physicians can now quickly and efficiently assess cardiac output and fluid responsiveness at a stage in the care of the critical patient in which goal-directed therapy is most crucial. A familiarity with these advanced techniques, as well as their appropriate application and limitations, will assist the emergency physician in meeting resuscitative endpoints.
LITERATURE TABLE
CI, confidence interval.
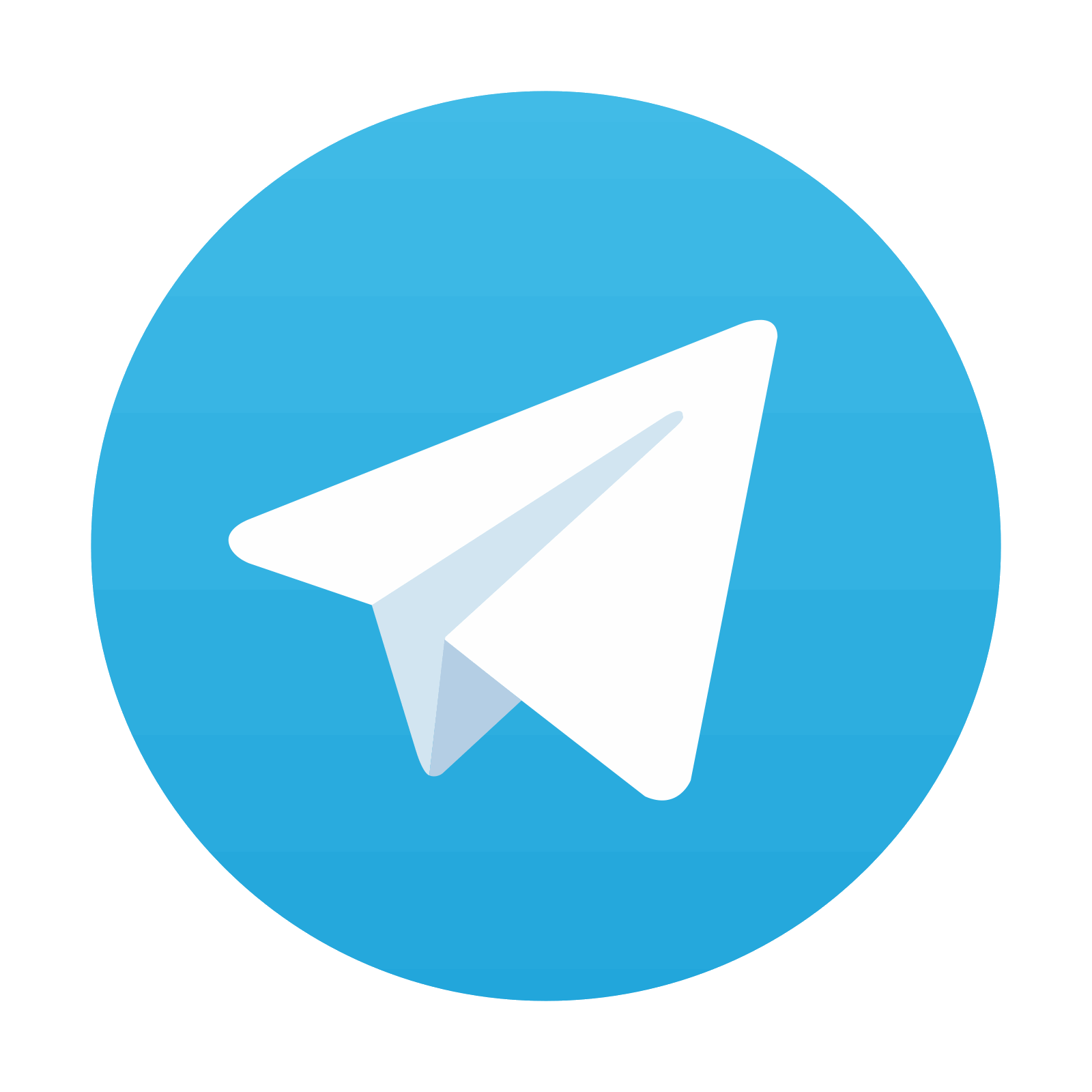
Stay updated, free articles. Join our Telegram channel
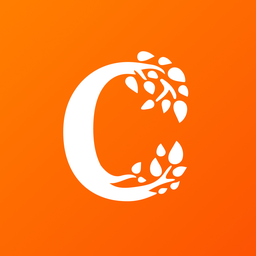
Full access? Get Clinical Tree
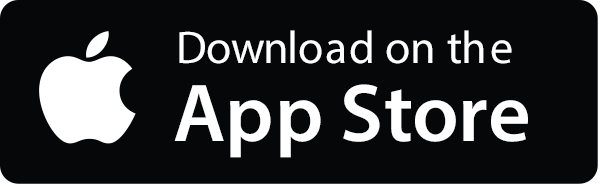
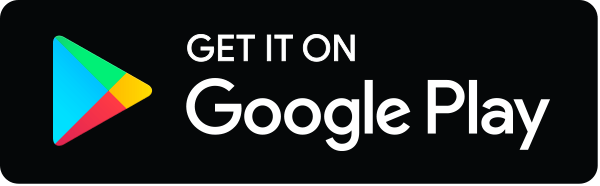