Chapter Outline
EXOGENOUS MODES OF NEUROSTIMULATION
SITES OF STIMULATION AND ASSESSMENT
MONITORING OF NEUROMUSCULAR BLOCKADE AND RECOVERY
Visual and Tactile Assessment of Evoked Responses
ELECTROMYOGRAPHY VERSUS MECHANOMYOGRAPHY
Overview
In the nearly 70 years since the introduction of curare to clinical anesthesia by Griffith and Johnson, neuromuscular blocking drugs (NMBDs) have emerged as agents that are vital to the care and well-being of patients undergoing anesthesia and surgery. However, there is still uncertainty regarding the best means to administer NMBDs and monitor their effects. Patients vary widely in their response to these drugs, necessitating careful titration for induction and maintenance of block and careful assessment of recovery. As a result of this wide interpatient variability, residual block or weakness (residual “curarization”) has been documented following a variety of regimens despite the intraoperative use of peripheral nerve stimulators.
Neuromuscular Physiology
Nerve Conduction
The motor neuron is a single nerve cell whose body is located in the ventral horn of the spinal cord. From here, its axon extends to the muscle fibers that it innervates. When the membrane potential of the motor nerve increases above threshold, an “all-or-none” action potential is generated and translated to the end plate of each muscle fiber by the release of acetylcholine (ACh) across a neuromuscular junction (NMJ). The number of muscle fibers innervated by a single motor neuron, known as the innervation ratio of the motor unit, determines the functional intricacy of the particular muscle. For example, where fine movements are required, such as with the extraocular (orbicularis oculi) or digital (lumbrical, interossei) muscles, the same neuron innervates only a few muscle fibers. This low innervation ratio allows very fine control of a single muscle group whose function is modulated by many neurons. In contrast, large muscle groups that do not require fine control over movement, such as the postural muscles of the back, have hundreds of muscle fibers innervated by only one neuron, or a high innervation ratio.
Neuromuscular Junction
The NMJ consists of the presynaptic region of the motor neuron, the motor end plate, and the intervening cleft ( Fig. 15-1 ). The region is enclosed by Schwann cells, which separate it from the surrounding tissues and extracellular fluid. The presynaptic terminal is an unmyelinated portion of the axon designed for the synthesis, storage, and release of ACh. The membrane is folded into many closely interposed longitudinal gutters that increase its surface area. Each vesicle appears to contain 5000 to 10,000 molecules of ACh. The ACh contained in a single vesicle often is referred to as a quantum of transmitter, which is capable of generating a miniature end plate potential (MEPP). Quanta are continuously released into the synaptic cleft by exocytosis. Excitation of the motor nerve, so-called motor nerve firing, causes the synchronized release of multiple quanta, the effects of which summate at the end plate to generate a muscle action potential (MAP).

The postsynaptic membrane, much like the presynaptic membrane, is folded to increase its surface area. The mature postsynaptic end plate contains nicotinic acetylcholine receptors (nAChRs) at a density of 10,000/μm 2 , each of which consists of five subunits—two α and one each of β, δ, and ϵ—arranged in a rosette ( Fig. 15-2 ). The recognition site of each of the two α-subunits must be occupied by ACh or an exogenous depolarizing agent (succinylcholine) for the all-or-none opening of a given channel to occur. Each open channel then allows the entry of Na + and Ca 2+ into the cell and allows the exit of K + . The end plate membrane serves as a resistor, such that the ion flux generates an end plate potential (EPP). Individual EPPs may accumulate and thus summate to reach threshold. When approximately 250,000 to 500,000 channels (5% to 10%) of a given NMJ are open, the summated EPP typically reaches the threshold required to elicit an MAP. This usually occurs in response to a synchronized release of quanta from the nerve terminal. The end plate response is short-lived because of rapid metabolism of ACh by acetylcholinesterases (AChEs) at the synaptic cleft. At the NMJ, AChE exists in the asymmetric, or A12, form, consisting of three tetramers of catalytic subunits covalently linked to a collagen-like tail. Approximately 50% of the released ACh is hydrolyzed during the time of diffusion across the synaptic cleft before reaching nAChRs. The efficiency of AChE depends on its rapid catalytic activity. In fact, AChE has one of the highest catalytic efficiencies known. It can catalyze ACh hydrolysis (4000 molecules of ACh hydrolyzed per active site per second) at near diffusion-limited rates.

The released ACh binds to α-subunits of the nAChRs. These ligand-gated cation channels allow sodium to enter and depolarize the muscle cell membrane at the NMJ. This depolarization activates voltage-gated sodium channels, which mediate the initiation and propagation of action potentials across the surface of the muscle membrane and into the transverse tubules (T-tubules), which results in the upstroke of the action potential.
There are two types of calcium channels: dihydropyridine receptor (DHPR) in the T-tubules and the ryanodine receptor (RyR1) in the sarcoplasmic reticulum (SR). The DHPRs act as voltage sensors and are activated by membrane depolarization. When the membrane is depolarized, the RyR1 receptors are activated. The DHPR-RyR1 interaction releases large amounts of Ca 2+ from the SR, causing muscle contraction. This process is known as excitation-contraction coupling. Repolarization of the muscle membrane is initiated by the closing of the sodium channels and by the opening of the potassium ion channel that conducts an outward K + current. The return of the muscle membrane potential to its resting level (approximately −70 to −90 mV) is achieved by allowing Cl − to enter the cell through voltage-sensitive chloride channels.
Neuromuscular Blocking Drugs
Both nondepolarizing and depolarizing relaxants cause flaccid paralysis by their actions at the NMJ. The characteristic patterns of these types of blocks are illustrated in Figure 15-3 . Nondepolarizing agents compete with ACh by competitively binding to the α-subunits of the receptor, which have a high affinity for the receptor but exhibit no appreciable agonist activity. Postsynaptic binding prevents the receptor channel from opening. Presynaptic binding opposes the mobilization and release of ACh. Studies with nondepolarizing blockers have demonstrated that when twitch height has returned to baseline, significant degrees of fade in the train-of-four (TOF) response may still be present.


It is generally believed that twitch depression results from block of postsynaptic nAChRs, whereas tetanic or TOF fade results from block of presynaptic nAChRs. Blockade of the presynaptic nAChRs by neuromuscular blockers prevents ACh from being made available—that is, it prevents its release from presynaptic nerve terminals—to sustain muscle contraction during high-frequency (tetanic or TOF) stimulation. Because the released ACh does not match the demand, fade is observed in response to stimulation. However, there is also strong contrary evidence indicating that fade could be simply a postjunctional (postsynaptic) phenomenon. This latter argument is supported by the fact that the snake α-bungarotoxin, which binds irreversibly to muscle (postjunctional) nAChRs but does not bind to neuronal (prejunctional) nAChRs, does produce fade. Although all nondepolarizing relaxants also bind presynaptically, some drugs such as curare, pancuronium, and rocuronium have an extremely high affinity for these receptors.
Depolarizing blockers bind with high affinity to junctional receptors and act as agonists (i.e., they are similar in structure to ACh) at postsynaptic nAChRs. They cause prolonged membrane depolarization that prevents generation of subsequent action potentials and hence results in neuromuscular block. An MAP can no longer be propagated, and flaccid paralysis ensues. Succinylcholine is the only depolarizing neuromuscular blocker currently in clinical use; it is hydrolyzed in plasma by butyrylcholinesterases (plasma cholinesterases) to succinylmonocholine and choline, such that only 10% of the administered drug reaches the NMJ. Succinylmonocholine is a much weaker neuromuscular blocking agent than succinylcholine and is metabolized much more slowly to succinic acid and choline. Because there is little or no butyrylcholinesterase at the NMJ itself, butyrylcholinesterase influences the onset and duration of action of succinylcholine by controlling the rate at which the drug is hydrolyzed in the plasma before it reaches, and after it leaves, the NMJ. Recovery from succinylcholine-induced blockade occurs as succinylcholine diffuses away from the NMJ, down a concentration gradient as the plasma concentration decreases.
In the case of administration of a depolarizing neuromuscular blocking agent, such as succinylcholine, the muscle response that has been classically described is quite different. Depolarizing block, also called phase I block, is often preceded by muscle fasciculation. During partial neuromuscular block, depolarizing block is characterized by 1) a decrease in twitch tension, 2) no fade during repetitive stimulation (tetanic or TOF), and 3) no posttetanic potentiation. However, with prolonged exposure of the NMJ to succinylcholine, or with administration of a single dose in patients with an abnormal genetic variant of butyrylcholinesterase, the characteristics of the neuromuscular block can be changed from those of the classic depolarizing (phase I) block to those of phase II block, characterized by fade during TOF and tetanic stimulation and posttetanic potentiation similar to that induced by nondepolarizing neuromuscular blockers. A recent study, however, demonstrated that posttetanic potentiation and fade in response to TOF and tetanic stimuli are characteristics of neuromuscular block even after a single bolus administration of different doses of succinylcholine. It seems that some characteristics of a phase II block are evident from an initial dose as small as 0.3 mg/kg of succinylcholine.
In addition to the classic nondepolarizing and depolarizing forms of neuromuscular block, the NMJ also may be inactivated by such phenomena as channel block and desensitization. Channel block entails direct occlusion of the receptor channels. Open-channel block, either use dependent or voltage dependent, involves occlusion of an otherwise open receptor channel, typically by a charged molecule. Closed-channel block involves occlusion independent of channel opening. Desensitization occurs when the receptor and its channel are not responsive to the presence of agonists on both α-subunits. This typically entails a reversible conformational change of variable duration as a result of persistent binding of depolarizing agents to the receptor. This persistent binding may change the typical (phase I) block of a depolarizing relaxant to one that assumes the characteristics of a nondepolarizing block (phase II). Long-term effects also may be a consequence of inactivation of cellular mechanisms by long, thin molecules such as decamethonium, which may enter the cytoplasm through the open receptors. Barbiturates, potent volatile anesthetics, receptor agonists, cholinesterase inhibitors, local anesthetics, phenothiazines, calcium channel blockers, antibiotics, alcohol, naltrexone, and naloxone all may cause conformational changes of the receptor and/or occlude the channel. All these may enhance or prolong the effects of an existing block from a muscle relaxant.
Exogenous Modes of Neurostimulation
In the clinical setting, neurostimulation typically is delivered by a 9-V battery-powered, adjustable direct current (DC) stimulator via subcutaneous needles or surface electrodes. The stimulus should be monophasic (i.e., square wave and unidirectional) because biphasic waves may produce repetitive stimulation. The other critical components of a stimulus are its pulse duration (in milliseconds) and intensity (in milliamperes). Pulse duration must be less than 0.5 ms, so as not to induce repetitive neural firing or direct muscle stimulation. The intensity of the stimulus should be sufficient to depolarize nerve fibers at the given pulse duration (i.e., supramaximal). This stimulus current should remain constant, with the output voltage varying automatically as skin resistance changes over time. Lastly, the stimulator should be able to produce multiple patterns of stimulation, such as single twitch, TOF, tetanic, and so forth. Optimally, a stimulator should have a rheostat for adjusting output current, and lead disconnect, excessive impedance, and low-battery indicators as well as a visual and/or audible stimulus-delivery indicator. The responses to changes in stimulus duration and intensity are illustrated in Table 15-1 , which compares mechanomyographic (MMG), electromyographic (EMG), and acceleromyographic (AMG) responses in unblocked, awake volunteers.
Current (mA) | Pulse Width (ms) | MMG (% Response) | EMG (% Response) | AMG (% Response) |
---|---|---|---|---|
20 | 0.10 | 0.8 | 1.0 | |
0.15 | 5.4 | 1.3 | ||
0.20 | 29.7 | 11.4 | 12.0 | |
0.30 | 29.0 | |||
0.40 | 67.6 | 55.8 | ||
40 | 0.10 | 13.5 | 1.5 | |
0.15 | 45.9 | 20.1 | ||
0.20 | 86.5 | 65.9 | 54.0 | |
0.30 | 79.0 | |||
0.40 | 97.3 | 102.6 | ||
50 | 0.20 | 80.0 | ||
0.30 | 101.0 | |||
60 | 0.10 | 40.5 | 5.1 | |
0.15 | 81.1 | 49.0 | ||
0.20 | 100.0 | 100.0 | 100.0 | |
0.30 | 119.0 | |||
0.40 | 102.7 | 121.1 | ||
70 | 0.10 | 48.6 | 8.2 | |
0.15 | 102.7 | 57.5 | ||
0.20 | 102.7 | 106.0 | ||
0.30 | ||||
0.40 | 108.1 | 126.0 |
∗ Values are expressed as percent of the value achieved with a stimulus amplitude of 60 mA and a stimulus duration (pulse width) of 0.2 ms.
When assessing the effect of NMBDs on the response to single stimuli over a period of time, the same number of nerve fibers must be stimulated. For this reason, anesthesiologists traditionally have relied on a supramaximal stimulating current—that is, a current that is 10% to 20% greater than the current needed to stimulate all the efferent fibers in the nerve bundle. Use of a supramaximal current allows current to vary slightly over time without affecting the total number of nerve fibers stimulated. This is readily achieved with needle electrodes, typically at less than 10 mA. Surface electrodes may fail to stimulate all fibers, especially when they are not in close proximity to the nerve, such as with improper electrode placement or in obese patients, even at currents of 50 to 70 mA. It is frequently stated that current intensity determines neural stimulation. In reality, the electrical charge (current [mA] × duration [ms] = microcoulombs [μQ]) is the essential element.
The current output of a stimulator must remain within a narrow range over time and over the range of physiologic resistances. Although delivery of a constant current can be accomplished most effectively with needle electrodes, insertion into the skin may be painful to the awake patient; surface electrodes are most commonly used in the clinical setting. Skin resistance typically is decreased by use of an electrolyte solution, such as silver/silver chloride. However, several minutes are required to allow for it to attain optimal effectiveness. The time for this electrode “curing” may be accelerated by cleansing and degreasing the skin, such as with alcohol or acetone, and by abrading the skin with an abrasive gel (NuPrep; ADInstruments, Colorado Springs, CO; Fig. 15-4 ).

Although rare, complications associated with the use of nerve stimulators can occur. Needle electrodes may be a source of local irritation, infection, and nerve damage because of intraneural placement. They also are more likely to be associated with local tissue burns from electrosurgical units because they provide good contact, with minimal resistance, for exit of high-frequency current over a small area of skin. In general, the use of needle electrodes in clinical practice is not recommended; however, if they are used, caution should be exercised to ensure that the needle is applied adjacent to the nerve, not through it.
Effect of Stimulus Frequency
In the setting of normal, unblocked neuromuscular transmission, increasing the rate of stimulation from single stimuli at 0.1 Hz (one every 10 seconds) to brief tetanic stimulation at 50 Hz results in sustained muscle contractions (tetanus) without fade. At supraphysiologic rates of stimulation (>70 to 200 Hz), even normal neuromuscular transmission may fatigue. When a nondepolarizing block is present, such fatigue is noted at slower rates of neurostimulation. This constitutes the basis for assessments of response to tetanic (at 50 Hz) and TOF (at 2 Hz) stimulation.
The rate of neurostimulation has pronounced effects on assessments of depth of block. In addition to promoting fatigue, frequent stimulation also results in as much as a fivefold to sixfold increase in local blood flow. This may result in more rapid delivery of relaxant to the stimulated muscle, especially if neurostimulation is initiated before administration of relaxant. Ali and Savarese reported that the apparent dose requirements for d-tubocurarine at the adductor pollicis muscle decreased by a factor of three as the stimulus frequency increased from 0.1 Hz to 1.0 Hz. Of perhaps even greater clinical significance is the effect of stimulus frequency on the apparent onset of blockade. Increasing the stimulus frequency results in an apparent greater degree of neuromuscular depression at the site of stimulation for both depolarizing and nondepolarizing block.
Patterns of Stimulation
Currently, several patterns of stimulation are used to assess the degree of neuromuscular block. These include single stimuli, tetanic stimulation, TOF, and double-burst stimulation (DBS) ( Fig. 15-5 ). The newer nerve stimulators are capable of functioning in any of these modes as well as in posttetanic count (PTC) mode ( Fig. 15-6 ).


Single Repetitive Stimulation: Single Twitch
This method consists of assessing the response to an individual stimulus or serial stimuli, usually at frequencies between 0.1 Hz (1 stimulus every 10 seconds) and 1.0 Hz (1 stimulus per second). The use of 1.0 Hz should be discouraged for reasons given above. Single-twitch (ST) stimulation is the least precise method of assessing partial neuromuscular blockade under clinical conditions, and it requires measurement of a baseline (no block) ST amplitude for comparison with subsequent responses. In addition, the clinically useful range of block is limited because the response to a single stimulus is not reduced until at least 75% to 80% of the receptors are blocked; the response disappears completely once 90% to 95% of the receptors are blocked. Furthermore, ST monitoring is highly sensitive to variations in stimulating current, temperature, and preload (i.e., resting muscle tension).
As previously noted, it is imperative that the stimulating current remain constant over time to ensure that the same number of nerve fibers reach threshold with each stimulation. By applying a current that is 10% to 20% above the level required to stimulate all fibers of the motor nerve (i.e., supramaximal stimulus), the impact of variables such as temperature, skin resistance, and changes in electrode conductance can be minimized. However, a supramaximal stimulus may not always be delivered when surface electrodes are used. Despite these limitations, the response to ST stimulation is the standard for comparisons of NMBD potency. The effective dose of a muscle relaxant required for 95% depression of the ST height is defined as ED 95 and is a measure of drug potency.
Train-of-Four Stimulation
TOF stimulation consists of four repetitive stimuli at a frequency of 2 Hz. As noted above, even this relatively slow rate of stimulation is associated with fade in the context of nondepolarizing block. TOF is the most commonly used method of neuromuscular assessment in clinical practice. It is far less painful to awake patients than tetanus (a sustained, rapid stimulus) and is not associated with prolonged posttetanic effects at the NMJ. In some cases, it may be more sensitive than tetanus to the presence of residual block. In the context of nondepolarizing block, both TOF and tetanus result in fatigue, which is manifested by fade. However, in contrast to tetanus, TOF does not increase or facilitate neuromuscular responses during and after application.
Unlike single stimuli, TOF stimulation does not require a prerelaxant baseline for comparison. It assesses the relationship among successive responses, thus serving as its own control. The relationship between the size of the fourth twitch response (T 4 ) and the first twitch response in the TOF (T 1 ) is expressed as T 4 /T 1 , or fade ratio. In the absence of nondepolarizing block, the T 4 /T 1 ratio is approximately 1.0. In the context of nondepolarizing block, decreases in this ratio depend on such factors as the relaxant used and whether monitoring is performed during onset or recovery. Typically at 70% to 75% receptor occupancy, T 4 will start to decrease selectively. At a T 4 /T 1 ratio as low as 0.70, T 1 may still be close to its baseline height. When T 4 is no longer detectable, either visually or mechanomyographically, T 1 is approximately 25% of its baseline size. This corresponds to a block of approximately 80% of the receptors. The third twitch response (T 3 ) is lost when approximately 85% of receptors are blocked, whereas the second (T 2 ) is lost when approximately 85% to 90% of receptors are blocked. During the remaining ST monitoring, the height of T 1 is progressively decreased, and T 1 is lost when 90% to 95% of receptors are blocked ( Table 15-2 ).
Total Receptors Blocked (%) | First Twitch (T 1 ) (% Normal) | Fourth Twitch (T 4 ) (% Normal) | T 4 /T 1 |
---|---|---|---|
100 | 0 | 0 | — |
90-95 | 0 | 0 | T 1 lost |
85-90 | 10 | 0 | T 2 lost |
20 | 0 | T 3 lost | |
80-85 | 25 | 0 | T 4 lost |
80-90 | 48-58 | 0.60-0.70 | |
95 | 69-79 | 0.70-0.75 | |
75 | 100 | 75-00 | 0.75-1.0 |
100 | 100 | 0.9-1.0 | |
50 | 100 | 100 | 1.0 |
25 | 100 | 100 | 1.0 |
In contrast to ST monitoring, TOF may also allow detection of a phase II block in response to a depolarizing agent. Normally, a depolarizing muscle relaxant causes a progressive decrease in the size of the ST or a symmetrical decrease in the size of all responses of the TOF or response to tetanus. Because no fade is present, the T 4 /T 1 ratio is maintained near 1.0 until all twitches disappear. However, when phase II block occurs, the depolarizing relaxant takes on features of a competitive (nondepolarizing) relaxant, and fade develops in response to TOF or tetanic stimulation. This type of block cannot be assessed by ST monitoring.
TOF stimulation provides other advantages as well. The T 4 /T 1 ratio is consistent at submaximal and at supramaximal stimulating current intensities, so long as T 1 and T 4 responses are detectable ( Fig. 15-7 ), especially at 10 mA or more above the T 4 threshold. Likewise, evidence suggests that although T 1 amplitude increases with both increase in stimulus duration and intensity, the TOF ratio of the evoked responses is independent of stimulus duration, so long as the pulse width is less than 0.5 ms ( Fig. 15-8 ). The consistency of the T 4 /T 1 ratio at varying current intensities and at varying stimulus durations facilitates testing of awake patients because discomfort is directly related to the intensity of the stimulating current.


Tetanic Stimulation
Tetanic (TET) stimulation consists of repetitive high-frequency neurostimulation (≥30 Hz). This results in repetitive MAPs and persistent muscle contraction. In the unblocked NMJ, each contraction can be sustained for several seconds at stimulating frequencies as high as 70 to 100 Hz. In clinical practice, a 5-second, 50-Hz TET stimulus is used most often because the evoked muscle tension approximates the tension developed during maximal voluntary effort.
Although normal muscle responds to TET stimulation with summated contractions that overcome elastic forces and produce an augmented response, the TET response fades, or fatigues, in the context of nondepolarizing block ( Fig. 15-9 ). Tetanic fade most likely represents a presynaptic inability to mobilize ACh rapidly enough to maintain depolarization despite repetitive nerve firing. It may be attributable to competition by nondepolarizing agents at presynaptic cholinergic receptors involved with Ca 2+ flux and ACh mobilization. The consequences of the diminished release of ACh are magnified by binding of the nondepolarizing agent to the postsynaptic receptors, thereby decreasing the margin of safety. Evidence also suggests that fade in response to TET stimulation may occur during the use of potent inhaled anesthetics in the absence of nondepolarizing block.

Another effect of a TET stimulus is the potential alteration of subsequent evoked neuromuscular responses. In the presence of a nondepolarizing NMBD, or phase II block with a depolarizing relaxant, stimulation after tetanus may result in posttetanic facilitation or potentiation. The magnitude of posttetanic effects on evoked twitch size is a function of depth of block. Testing of neuromuscular function within 2 to 5 minutes of a preceding TET stimulation can lead to overestimation of evoked responses, that is, underestimation of depth of block ( Fig. 15-10 ). Although this has been shown to be true for T 1 , TOF, and DBS, subsequent tetanic fade seems to be relatively immune to the phenomenon. One theory to explain posttetanic facilitation is that the intense tetanic stimulus induces an increase in the mobilization and subsequent release of ACh quanta, which results in an increase in subsequent EPPs. Another theory has suggested that tetanus may have relatively long-term effects on the NMJ by displacing relaxant from the postsynaptic receptor. In either case, posttetanic stimulation during nondepolarizing blockade results in generation of a greater EPP than that obtained before tetanus.

Posttetanic Count
When the use of nondepolarizing NMBDs results in 100% twitch-size depression, the potentiation that occurs following TET stimulation may enable detection of the response to single stimuli. The number of twitch responses elicited by serial stimulation at 1 Hz (beginning 3 seconds after a 5-second, 50-Hz tetanus) is inversely related to the depth of block: the greater the number of posttetanic responses, the less the degree of block and the more rapid the ensuing recovery (see Figs. 15-6 and 15-10 ; Table 15-3 ). This method of assessment also may be indicated to ensure profound paralysis; that is, ablation of PTC as well as response to twitch and tetanus. It also provides an indication as to when recovery of ST may be anticipated and thus provides a guide for planning reversal of residual block with anticholinesterases.
Posttetanic Counts ∗ | Atracurium Time (Min) | Pancuronium Time (Min) |
---|---|---|
1 | 9 | 35 |
2 | 7 | 28 |
4 | 4 | 20 |
6 | 2 | 12 |
8 | 0-2 | 6 |
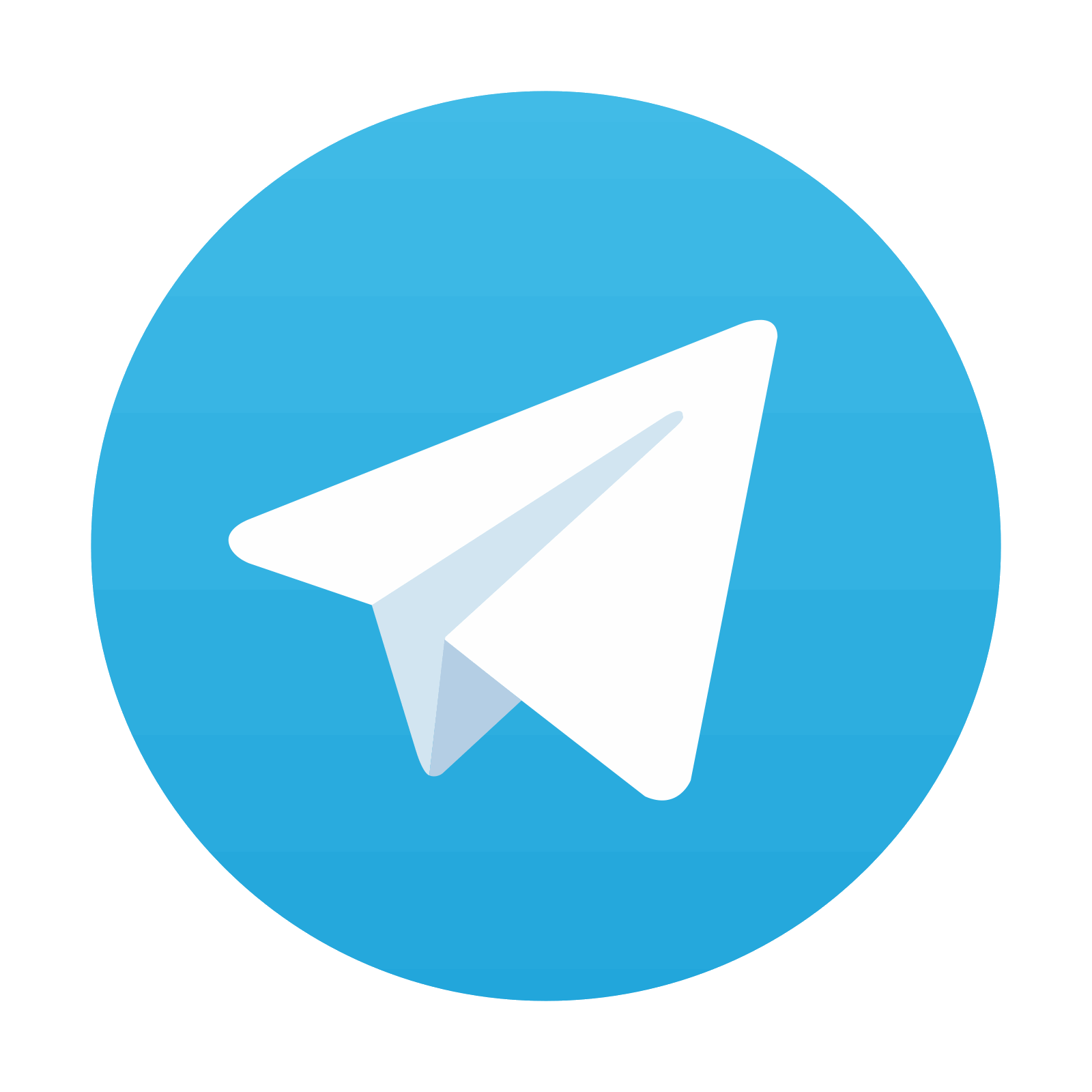
Stay updated, free articles. Join our Telegram channel
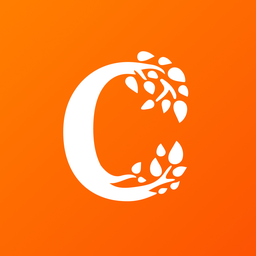
Full access? Get Clinical Tree
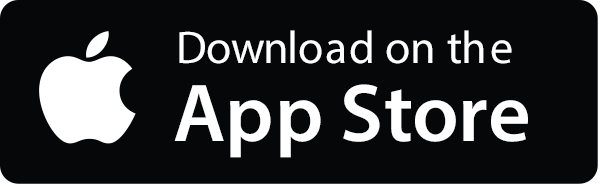
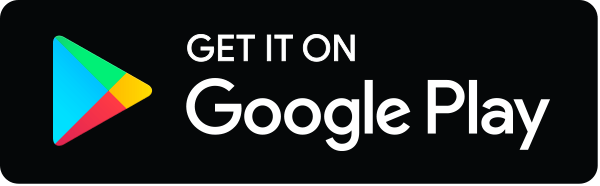
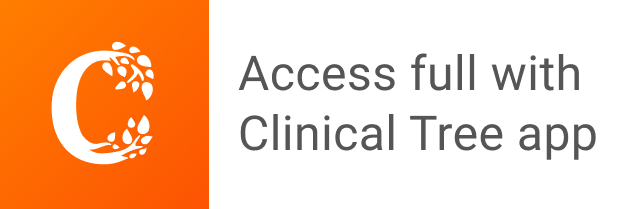