Pearls
- •
Workload and efficiency define the demands that disease imposes on the respiratory muscles and therefore are the relevant variables in the analysis of the mechanical function of the respiratory system.
- •
The work done by the respiratory muscles and, by extension, the energy that must be supplied to these muscles are defined by the combined volume-pressure relationships of the lungs and chest wall.
- •
Volume changes within the respiratory system are dictated primarily by the body’s need to take up oxygen and eliminate carbon dioxide and therefore are determined by factors such as physical activity or metabolic rate, which are relatively independent of the condition of the lungs and chest wall. Pressure changes, on the other hand, depend on physical processes that take place in the respiratory system’s components.
- •
The efficiency of the respiratory pump is influenced by factors that the astute clinician must consider when assessing a child’s ability to sustain an increased respiratory workload. These include the configuration of the diaphragm, presence of rib cage distortion, and nutritional state and conditioning of the respiratory muscles.
- •
It is a common clinical observation that different types of mechanical derangement result in distinctive patterns of breathing. These patterns generally agree with the principle of minimal power expenditure and can be useful to categorize the type of derangement the patient has during the initial evaluation.
Much like the cardiovascular system, the lungs and chest wall can be likened to a mechanical pump that draws in air from the atmosphere into the alveoli and pushes it back into the atmosphere. To generate the required pressure gradients, it is necessary to overcome the resistance and elastance of the thorax, airways, and lungs. During spontaneous tidal respiration, the lungs and chest wall can be considered as two sets of springs in a stretched position that are exerting their recoil, under normal circumstances, in opposite directions. While the chest wall is powered by muscles for inflation and deflation, the lungs operate predominantly with their inherent elastic recoil for generation of pressure. Just as failure of the cardiac pump can be treated with mechanical support, the respiratory pump is also amenable to extracorporeal support to address the specific alterations responsible for its mechanical dysfunction.
Unlike the cardiac pump, the respiratory machine does not have an intrinsic pacemaker. It is driven by a central controller (autonomic and voluntary) located in the brain, which receives feedback from a variety of sensors located centrally and peripherally in the form of chemoreceptors and mechanoreceptors. The controllers, with the input from the sensors, drive the respiratory machine, employing its effectors —consisting of the diaphragm and intercostal and abdominal muscles. The effectors create the necessary pressure gradients, which act on the targets (conducting airways and gas exchange elements) to generate airflow and move volumes of gas by overcoming airway/tissue resistance and pulmonary/thoracic elastance. Mechanical dysfunction can impede airflow (resistive dysfunction) or prevent tissue expansion (restrictive dysfunction).
The efficiency of the mechanical pump in moving air across the airways and alveoli may be compromised by abnormalities of any one of the effectors or target structures, either individually or in combination. A keen understanding of the functioning of individual components of the machine is necessary to address its failure.
Pump dysfunction and failure
Pressures
Pressure generation is a requirement for air movement to occur in the respiratory system. However, some disagreement and confusion exist within scientific literature regarding the precise definitions of pressures within the respiratory system. Proper understanding of these pressures is essential to understanding respiratory function.
Fig. 46.1 represents the important pressures generated in the respiratory system. Pressure at the airway opening (P AW ) during spontaneous unassisted respiration is atmospheric pressure (P ATM ) and usually taken as zero (cm H 2 O) relative to other pressures generated. In mechanically ventilated patients, P AW refers to the pressure at the patient-ventilator interface, such the tracheal tube or the face mask. The transrespiratory pressure (P TR ) is P AW − P ALV (alveolar pressure) and is responsible for generating airflow. With no air movement, such as at end-inspiration or end-expiration, the P TR is zero, meaning that P AW is equal to P ALV . As spontaneous inspiration begins, pleural pressure (P PL ) decreases due to contraction of the respiratory muscles and expansion of the chest wall. This negative P PL is transmitted to all structures inside the chest, including the airways and alveoli, so that a pressure gradient for air movement develops. As P ALV increases due to air entry and matches P AW , airflow stops. During exhalation, P ALV becomes positive and the process reverses as air flows from high pressure in the alveoli out the airway opening until P ALV equals P AW again. P PL is rarely measured directly but can be inferred using a distal esophageal pressure catheter (P ES ) and is negative during unassisted breathing. Likewise, P ALV cannot be measured directly but can be estimated using airway occlusion techniques. After airway occlusion, airflow ceases and the P ALV equilibrates with P AW , which can be measured. Transpulmonary pressure (P TP ) is the pressure gradient between P ALV and P PL and is indicative of the stress experienced by the alveolar walls. Transthoracic pressure (P TT ) is defined as the pressure between the P PL and P ATM . P TT is used to calculate thoracic cage compliance.

Lung volumes and capacities
Along with pressure, understanding lung volumes and capacities is crucial to understanding normal lung function and many pathologic conditions ( Fig. 46.2 ). Tidal volume (V t ) is the volume of gas moved with each breath. In health, spontaneous V t is usually between 6 and 7 mL/kg. This volume refreshes alveolar gas during inspiration and leads to removal of carbon dioxide (CO 2 ) during exhalation. The volume of gas remaining in the lung after tidal respiration is termed functional residual capacity (FRC). FRC acts as a reservoir for gas exchange between alveoli and pulmonary capillary blood throughout respiration. Diseases that decrease lung compliance and lower FRC can have profound effects on oxygenation. Positive end-expiratory pressure (PEEP) helps maintain end-expiratory volume and improve oxygenation. Residual volume (RV) is the volume of gas remaining in the lung after a maximal forced exhalation. The difference between FRC and RV is the expiratory reserve volume. Inspiratory capacity (IC) is the volume that can be inspired from FRC after a maximal inspiration and total lung capacity is the total volume of gas in the lung at maximum inspiration. Closing capacity (CC) is the volume of gas in the lung during exhalation when the dependent airways start to close. CC can be measured only by specific gas dilution techniques and not by spirometry. In healthy children and adults, CC is well below the FRC, meaning that all airways remain open during tidal respiration. In intrapulmonary obstructive diseases and even in healthy neonates, dependent airways start to close during tidal exhalation before reaching FRC. , Thus, alveolar ventilation below CC moves toward the nondependent, less perfused areas, away from dependent and better perfused areas, resulting in ventilation/perfusion (V/Q) mismatch and a lower partial pressure of arterial oxygen (Pa o 2 ). This also results in some amount of air trapping.

Flow/volume relationships
Flow/volume relationship curves are clinically useful tools for demonstrating the effect that changes in pulmonary mechanics have on volumes and gas flow ( Figs. 46.3 and 46.4 ). These curves are generated with spirometry machines and can be used in both the outpatient setting and at the bedside. Typically, a maximal inspiration is followed by maximal forced exhalation, generating a flow/volume loop. Forced vital capacity (FVC) is the total volume exhaled during this maneuver. Forced expiratory volume during the first second of exhalation is termed FEV 1. Decreases in FVC and FEV 1 result from decreased lung compliance, increased airway resistance, and decreased respiratory muscle strength. The maximum flow occurs in the first phase of forced exhalation and is referred to as FEF max (also referred to as peak flow ). It is effort dependent but also a marker of airway obstruction. The volume exhaled between 25% and 75% of the expiratory volume (FEF 25%–75% ) is relatively effort independent; decreases in this are suggestive of intrathoracic airway obstruction, such as in asthma (see Fig. 46.4 ). The shape of the expiratory curve gives clues to disease pathophysiology, as in obstructive disease, for which the mid-expiratory curve may be increasingly concave.


Equation of motion
As with any pump, the respiratory system function is determined by the physical properties of its components. The pressure necessary to move air into and out of the lungs is determined by two factors: (1) the elastance of the lung and chest wall and (2) airway resistance. The equation of motion describes the pressure gradient required to overcome these two factors ( Fig. 46.5 ). The pressure gradient required to overcome the elastic properties of the lung and chest wall and to effect volume change represents the force necessary to overcome the static properties of the respiratory system and is measured once flow has ceased. Additionally, the pressure required to create flow and move a certain amount of volume in a given time is a dynamic process that is determined by the resistive properties of the system and measured while flow is occurring. Together, these are the primary forces that govern respiratory mechanics.

Elastance of a tissue is the tendency of that tissue to resist deformation. It is defined as the change in pressure applied to the system divided by the change in volume that results from this change in pressure (ΔP/ΔV). The pressure needed to overcome tissue elastance is a static property of the system and is measured when airflow has ceased. Elastic recoil pressure is the pressure generated as the tissue returns to its resting state once the deforming stress (pressure) ceases. Elastic recoil of the respiratory system increases as lung volume increases above FRC.
At end-exhalation, the lungs tend to recoil inward toward a lower volume and the chest wall tends to recoil outward toward a higher volume. The balance of these forces determines FRC. The primary determinants of lung recoil pressure are elastin content within the tissue and surface tension within the airspaces. The chest wall recoil is determined by the integrity and maturity of the ribs, along with the mass and inherent tone of the muscles of respiration. Although the lung and the chest wall have separate and opposite recoil pressures, they are linked via the pleural space and need to be considered together under most clinical situations.
The recoil pressure of the lung is relatively constant per unit volume over the developmental spectrum. However, the chest wall compliance (expansibility) changes significantly throughout development ( Fig. 46.6 ). At birth, chest wall compliance is increased owing to a decrease in elastic elements. This is primarily due to lack of ossification of the ribs. Based on lung and chest wall recoils alone, FRC should be much lower in the newborn. However, the actual FRC measured is remarkably similar to older children when corrected for size. This is because neonates, unlike older children, maintain inherent tone in the muscles of respiration at end-exhalation. This prevents exhalation to a lower FRC that would otherwise occur if driven by chest wall elastic recoil alone. Additionally, the higher respiratory rates and shorter expiratory times of neonates prevent cessation of flow during exhalation and result in incomplete emptying of alveoli. Also, neonates have a greater CC, which results in some amount of air trapping. By 1 year of age, chest wall elastance has increased sufficiently to maintain adequate FRC without the need of increased muscular tone at end-exhalation.

The implications of these developmental differences are apparent when considering states that decrease respiratory muscle tone, such as rapid-eye-moment sleep or pharmacologic muscle relaxation. In young infants, these states may have a significant effect on FRC and overall oxygenation due to lack of end-expiratory muscle tone. Similarly, disease states that alter level of consciousness or affect neurologic tone or muscular strength (e.g., myopathies or neuropathies) will have similar effects. It has been shown that during anesthesia and muscle relaxation, the FRC of a neonate may decrease by more than 50% in contrast to an adult in whom it declines by only 10% to 25%. Adverse effects of anesthesia on lung function are well described. These effects are even more pronounced in young children compared with adults.
Compliance is the opposite of elastance and can be considered as the distensibility or stretchability of a tissue. It is defined as the change in volume of a tissue divided by the change in pressure (ΔV/ΔP). In health, the respiratory system is relatively compliant, meaning that it does not require high pressures to expand. As compliance worsens (or elastance increases) in disease, more pressure is necessary to expand the lungs.
The forces of the lungs that determine elastic recoil primarily involve surface forces at the gas-liquid interface and, to a lesser extent, elastic tissue forces. Surface tension is a primary determinant of recoil pressure of the lungs and is defined as the pressure exerted by liquid at the air-liquid interface along the lining of the alveoli. Liquid molecules at this interface are attracted to themselves to a much greater degree than to the adjacent air. This creates tension within the alveoli and a natural tendency for them to collapse as the liquid lining its surface is drawn toward itself. This property was first identified in the lung in the 1920s, when it was shown that a lung completely filled with fluid instead of air has a much lower elastance than lungs filled with air.
As alveoli become smaller, the liquid layer along their surface becomes thicker and the attraction force among the water molecules becomes greater. As this occurs, surface tension increases. The pressure developed can be predicted using the Laplace equation, which describes the pressure inside a liquid bubble. This pressure is directly related to the surface tension of the liquid and inversely related to the radius:
As the radius decreases, pressure increases as the liquid film lining the alveoli becomes thicker and more water molecules interact. When considering individual alveoli, those that are smaller tend to collapse more than those that are larger in diameter ( Fig. 46.7 ). This represents an unstable condition in which alveoli that are smaller would keep getting smaller and empty into low-pressure larger alveoli. In intact lungs, this does not occur for two reasons: the action of surfactant and the interdependence of alveoli.

Surfactants are phospholipids and proteins that decrease surface tension of liquids. They significantly reduce surface tension in the lung by reducing the attraction of the water molecules along the air-liquid interface. Surfactant is produced in the type II alveolar cells and released onto the alveolar surface in response to alveolar stretch. The phospholipids align along the interface with their hydrophobic tails facing the alveolar cavity and their hydrophilic heads embedded in the liquid layer. As alveolar volume increases, the surfactant concentration at the interface decreases as it is spread over a larger surface area, and its concentration increases as alveolar volume falls. With increasing concentration, the surfactant effect of lowering surface tension increases. This allows smaller alveoli to maintain the same cavitary pressure as larger alveoli despite their lower volume, helping to prevent a further decrease in volume.
Although the function of surfactant has helped explain lung compliance and alveolar patency and has improved our understanding of lung function in health and disease for decades, the properties of a single alveolus and the law of Laplace oversimplify the forces acting on the intact lung, as alveoli are not independent of each other. Alveolar interdependence is thought to be another important factor in determining alveolar patency. As alveoli are adjacent and connected to each other, they exert tension on each other, and their interconnections tend to stabilize groups of alveoli. As the volume of a single alveolus decreases, the recoil forces of adjacent alveoli pulling it back open will increase, helping to maintain its patency.
In addition to the surface properties, the elastic elements of the tissues involved also help determine overall respiratory compliance. The interstitial components—such as connective tissue elastin, collagen, and other fibrous tissue—usually do not contribute to lung recoil at low lung volumes or FRC. At higher lung volumes, these tissues begin to add to recoil pressure as they become stretched. With age, these tissues become less elastic and can contribute to increased lung compliance. Interstitial fluid and pulmonary blood volume also contribute to lung recoil, increasing elastance. As interstitial fluid increases, such as in states of capillary leak or diseases of increased capillary hydrostatic pressure (e.g., heart failure), lung compliance will decrease.
Resistance is a dynamic property of the respiratory system and is defined as the amount of pressure necessary to generate a given amount of flow (volume/time). It is calculated as R = ΔP/Δflow (Ohm’s law) and is expressed as cm H 2 O/L per second. Resistance is directly proportional to the pressure gradient driving gas flow and inversely proportional to the rate of flow. When pressure gradient is zero and flow ceases, resistance is zero. Because resistance exists only while flow is occurring, it is often termed flow resistance .
Resistive forces
Although tissue (viscous) resistance is a component of total pulmonary resistance, it is relatively minor compared with airway resistance. The contribution of viscous resistance and tissue inertia is more important in neonates and in conditions with increased alveolar/interstitial fluid.
Resistance encountered by the respiratory system during air movement is determined primarily by airway diameter and flow dynamics. Laminar flow occurs when gas molecules are moving parallel to each other. Laminar flow travels through a tube as a series of concentric layers of gas, the outermost of which travels the slowest owing to friction with the walls of the tube, and the innermost travels the fastest ( Fig. 46.8 ). When flow is laminar, movement is primarily determined by the gradient in pressure, with higher gradients producing increased flow. Turbulent flow is the loss of this parallel arrangement, which occurs when the airways become acutely narrowed or at branch points or with sudden change in velocity, such as at airway junctions or bends.

Laminar flow can be predicted using the Hagen-Poiseuille law, which states:
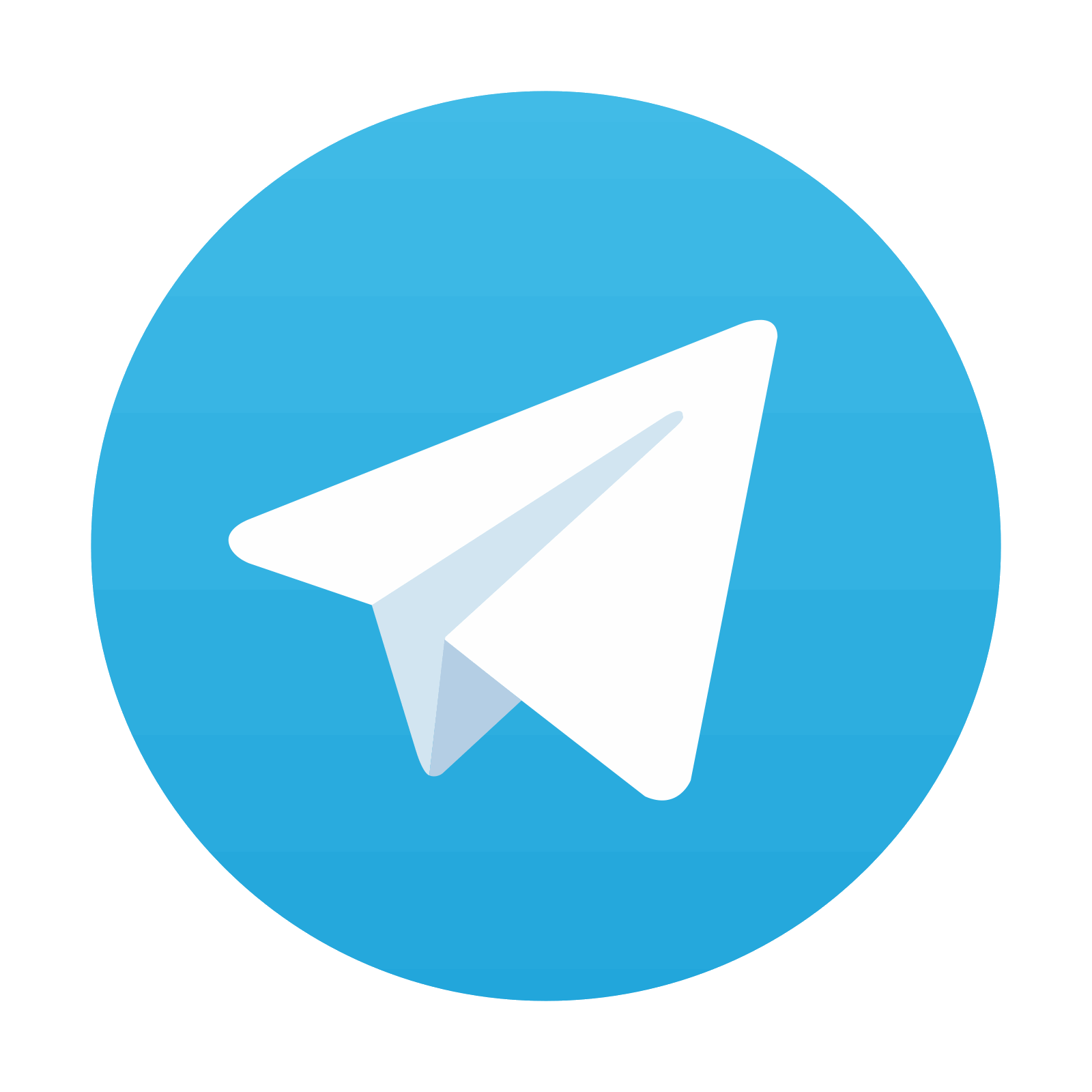
Stay updated, free articles. Join our Telegram channel
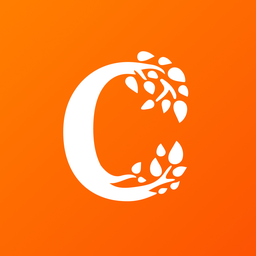
Full access? Get Clinical Tree
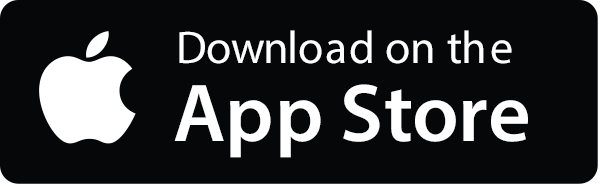
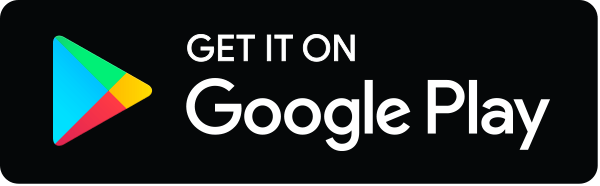
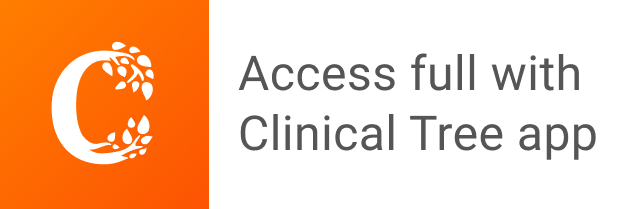