TABLE 18-1 DESIRABLE CHARACTERISTICS OF AN INTRAVENOUS ANESTHETIC
Stable in solution
Absence of pain on injection (venoirritation) or tissue damage from extravasation
Low potential to release histamine
Rapid onset
Prompt metabolism to inactive metabolites
Efficient clearance and redistribution mechanisms
Benign cardiovascular and ventilatory effects
Decrease in cerebral blood flow and metabolism
Prompt and complete return of consciousness
Absence of adverse postoperative effects (e.g., nausea, vomiting, delirium, headache)
FIGURE 18-2. A model depicting the postsynaptic receptor sites for the inhibitory neurotransmitter γ-aminobutyric acid (GABA) and the excitatory neurotransmitter glutamate in the central nervous system. When GABA occupies the receptor, it allows inward flux of chloride ions, resulting in hyperpolarization of the cell membrane and subsequent resistance of the neurons to stimulation by excitatory neurotransmitters. Barbiturates, benzodiazepines, and possibly propofol and etomidate decrease neuronal excitability by enhancing the effect of GABA at this receptor complex. When glutamate occupies the binding site of N-methyl-D-aspartate (NMDA) subtype of the glutamate receptor, the channel opens and allows sodium, potassium, and calcium ions to enter or leave the cell. Flux of these ions leads to depolarization of the postsynaptic neuron and initiation of an action potential. Ketamine blocks these open channels, inhibiting the excitatory response to glutamate.
B. Pharmacokinetics and Metabolism
1. The rapid onset of the CNS effect of most IV anesthetics can be explained by their high lipid solubility and relatively high cerebral blood flow (CBF).
2. The pharmacokinetics of IV hypnotics are characterized by rapid distribution and subsequent redistribution into several hypothetical compartments, followed by elimination (Table 18-2).
a. The primary mechanism for terminating the CNS effects of drugs used for the IV induction of anesthesia is redistribution from a central highly perfused compartment (the brain) to larger and well-perfused peripheral compartments (muscle, fat).
b. Most IV anesthetic agents are eliminated via hepatic metabolism (some metabolites are active) followed by renal excretion of more water-soluble metabolites.
c. For most drugs, the hepatic enzyme systems are not saturated at clinically relevant drug concentrations, and the rate of drug elimination decreases as an exponential function of the drug’s plasma concentration (first-order kinetics).
d. High steady-state plasma concentrations are achieved with prolonged infusions, hepatic enzyme systems can become saturated, and the elimination rate becomes independent of the drug concentration (zero-order kinetics).
TABLE 18-2 PHARMACOKINETIC VALUES FOR INTRAVENOUS ANESTHETIC DRUGS
e. Perfusion-limited clearance describes hepatic clearance of drugs (etomidate, propofol, ketamine, midazolam) in which extraction largely depends on delivery to the liver. (Hepatic blood flow decreases with upper abdominal surgery and increasing age.)
3. The elimination half-time (T1/2β) is the time required for the plasma concentration to decrease 50%.
a. Wide variations in T1/2β reflect differences in volume of distribution (Vd), clearance, or both.
b. Careful titration of an anesthetic drug to achieve the desired clinical effect is necessary to avoid drug accumulation and the resultant prolonged CNS effects after the infusion has been discontinued.
4. Context-sensitive half-time is the time necessary for the plasma concentration to decrease 50% in relation to the duration of the infusion. (This is important for determining recovery after varying length infusions of sedative–hypnotic drugs.)
5. Many factors contribute to interpatient variability in the pharmacokinetics of IV sedative–hypnotic drugs (Table 18-3).
C. Pharmacodynamic Effects
1. The principal pharmacologic effect of IV anesthetics is to produce dose-dependent CNS depression (dose-response curves) manifesting as sedation and hypnosis (Fig. 18-3).
TABLE 18-3 FACTORS THAT CONTRIBUTE TO INTERPATIENT VARIABILITY IN PHARMACOKINETICS
Degree of protein binding
Efficiency of renal and hepatic clearance mechanisms
Aging (lean body mass decreases)
Pre-existing diseases (hepatic, renal, cardiac)
Drug interactions
Body temperature
2. When steady-state plasma concentrations are achieved, it can be presumed that the plasma concentration is in equilibrium with the effect-site (receptor) concentration.
a. Efficacy of an IV anesthetic relates to the maximum effect that can be achieved with respect to some measure of CNS function (e.g., benzodiazepines are less efficacious than barbiturates in depressing electrical activity in the brain).
FIGURE 18-3. Dose–response relationships for sedation with midazolam and diazepam. The level of sedation was rated as 2 (awake and alert) to 6 (asleep).
b. Potency relates to the quantity of drug necessary to obtain the maximum CNS effect.
3. Most sedative–hypnotic drugs (except for ketamine) cause a proportional reduction in cerebral metabolism (CMRO2) and CBF, resulting in a decrease in intracranial pressure (ICP).
a. On the electroencephalogram (EEG), it is likely that whereas sedative doses produce activation of high-frequency activity, anesthetic doses produce a burst-suppression pattern.
b. Most sedative–hypnotic drugs can cause occasional EEG seizure-like activity despite also acting as anticonvulsants. One should differentiate between the epileptogenic activity (methohexital) and myoclonic-like phenomena (etomidate). Myoclonic activity is considered to be the result of an imbalance between excitatory and inhibitory subcortical centers owing to unequal degrees of suppression of these centers by the hypnotic drug.
4. Most sedative–hypnotics (the exception is ketamine) lower intraocular pressure in parallel with effects on ICP and blood pressure.
5. With the exception of ketamine (and to a lesser extent, etomidate), IV anesthetics produce dose-dependent depression of ventilation (transient apnea followed by decreased tidal volume).
6. Many different factors contribute to the hemodynamic changes associated with IV induction of anesthesia (Table 18-4).
TABLE 18-4 FACTORS THAT CONTRIBUTE TO THE HEMODYNAMIC EFFECT OF INTRAVENOUS INDUCTION
Drugs
Blood volume
Sympathetic nervous system tone
Speed of injection
Cardiovascular drugs
Preanesthetic medication
Direct effects on cardiac contractility or peripheral vasculature
7. The effects of IV anesthetics on neuroendocrine function are also influenced by surgical stimuli (release of vasopressin and catecholamines, decreased glucose tolerance).
8. Most IV sedative–hypnotic drugs lack intrinsic analgesic activity (except ketamine, which has pronounced analgesic-like activity).
D. Hypersensitivity (Allergic) Reactions
1. Allergic reactions to IV anesthetics or their solubilizing agents, although rare, can be life threatening.
2. With the exception of etomidate, all IV induction drugs have been alleged to cause some histamine release.
3. Although propofol does not normally trigger histamine release, life-threatening allergic reactions have been reported, especially in patients with a history of allergy to other drugs (most often muscle relaxants).
4. Barbiturates may precipitate episodes of acute intermittent porphyria in vulnerable patients. (The benzodiazepines, ketamine, etomidate, and propofol are reported to be safe.)
II. COMPARATIVE PHYSIOCHEMICAL AND CLINICAL PHARMACOLOGIC PROPERTIES
A. Barbiturates
1. Thiopental and thiamylal are thiobarbiturates with similar potency (adult induction dose, 3–5 mg/kg IV) and pharmacologic profile. Methohexital is an oxybarbiturate with a greater potency (adult induction dose, 1.5 mg/kg IV) than the thiobarbiturates and is associated with a high incidence of myoclonic-like muscle tremors and other signs of excitatory activity (e.g., hiccoughing).
a. These drugs are provided as racemic mixtures that are alkaline (thiopental 2.5% has a pH >9) and precipitate when added to acidic solutions (Ringer’s lactate).
b. Geriatric patients require a 30% to 40% reduction in the usual adult dose because of a decrease of the volume of the central compartment and slowed redistribution of thiopental from the vessel-rich tissues to lean muscle.
c. Thiopental is seldom used to maintain anesthesia because of its long context-sensitive half-time and prolonged recovery period.
d. Accidental intra-arterial injection of barbiturates may result in formation of crystals in arterioles and capillaries, causing intense vasoconstriction, thrombosis, and even tissue necrosis. (This is treated with intra-arterial administration of papaverine and lidocaine, brachial plexus block, and heparin.)
2. Barbiturates produce a maximum decrease in CMRO2 (55%) when the EEG becomes isoelectric (associated with a decrease in CBF and ICP).
a. An isoelectric EEG can be maintained with a thiopental infusion rate of 4 to 6 mg/kg/hr IV.
b. Although barbiturate therapy is widely used to control ICP after brain injury, the results of outcome studies are no better than with other aggressive forms of cerebral antihypertensive therapy.
c. Barbiturates have no place in the therapy after resuscitation of a cardiac arrest patient.
d. Barbiturates may improve the brain’s tolerance to incomplete ischemia and may be used for cerebroprotection during carotid endarterectomy, profound controlled hypotension, or cardiopulmonary bypass. Moderate degrees of hypothermia (33°C–34°C) may provide superior neuroprotection without prolonging the recovery phase.
e. Barbiturates possess potent anticonvulsant activity. Methohexital produces epileptogenic effects in patients with psychomotor epilepsy.
3. Barbiturates cause dose-dependent depression of ventilation. Bronchospasm and laryngospasm are usually the result of airway manipulation in the presence of inadequate anesthesia.
4. Cardiovascular effects of barbiturates include decreases in blood pressure decreased venous return because of peripheral pooling and direct myocardial depression and a compensatory increase in heart rate.
5. Hypotension is exaggerated in the presence of hypovolemia.
B. Propofol
1. As an alkylphenol compound, this drug is virtually insoluble in water, requiring its preparation in an egg–lecithin emulsion as a 1% (10 mg/mL) solution.
2. Propofol is rapidly cleared from the central compartment by hepatic metabolism, and the context-sensitive half-time for continuous IV infusions (≤8 hours) is <40 minutes. Emergence and awakening are prompt and complete even after prolonged infusions.
a. Hepatic metabolism is prompt to inactive water-soluble metabolites that are eliminated by the kidneys.
b. The clearance rate (1.5–2.2 L/min) exceeds hepatic blood flow, suggesting that an extrahepatic route of elimination (i.e., through the lungs) also contributes to its clearance (this is important during the anhepatic phase of liver transplantation).
3. The induction dose in adults is 1.5 to 2.5 mg/kg IV, and the recommended IV infusion rate is 100 to 200 μg/kg/min for hypnosis and 25 to 75 μg/kg/min for sedation. In the morbidly obese patient, the propofol induction and maintenance doses should be calculated based on the patient’s lean body weight.
a. Pain on injection occurs in a high proportion of patients when the drug is injected into small hand veins; this can be minimized by injection into larger veins or by prior administration of 1% lidocaine.
b. Propofol may produce a subjective feeling of well-being and euphoria and may have abuse potential as a result of these effects.
4. Propofol decreases CMRO2, CBF, and ICP, but the associated decrease in systemic blood pressure may also significantly decrease cerebral perfusion pressure.
a. Cortical EEG changes produced by propofol resemble those of thiopental.
b. A neuroprotective effect may reflect antioxidant properties.
c. Induction of anesthesia with propofol is occasionally accompanied by excitatory motor activity (nonepileptic myoclonia).
d. This drug is an anticonvulsant. The duration of seizure activity after electroconvulsive therapy is shorter with propofol than methohexital and is effective in terminating status epilepticus.
5. Propofol produces dose-dependent depression of ventilation. Apnea occurs in 25% to 35% of patients after induction of anesthesia.
a. Bronchodilatation may occur in patients with chronic obstructive pulmonary disease.
b. Hypoxic pulmonary vasoconstriction is not inhibited by propofol.
6. Propofol produces greater cardiovascular depressant effects than thiopental, reflecting decreased systemic vascular resistance (arterial and venous dilation) and direct myocardial depressant effects.
7. Propofol appears to possess antiemetic properties that contribute to a low incidence of emetic sequelae after propofol anesthesia. Subanesthetic doses (10–20 mg) may be used to treat nausea and emesis in the early postoperative period. Postulated antiemetic mechanisms include an antidopaminergic activity and a depressant effect on the chemoreceptor trigger zone and vagal nuclei.
8. Propofol decreases the pruritus associated with neuraxial opioids.
9. Propofol does not trigger malignant hyperthermia and may be considered the induction drug of choice in patients who are susceptible to malignant hyperthermia.
C. Benzodiazepines
1. The benzodiazepines of primary interest to anesthesiologists are diazepam, lorazepam, and midazolam and the antagonist flumazenil.
a. These drugs are primarily used as preoperative medication and adjuvant drugs because of their anxiolytic, sedative, and amnestic properties.
b. Diazepam and lorazepam are insoluble in water, and their formulation contains propylene glycol, a tissue irritant that causes pain on injection and venous irritation.
c. Midazolam is a water-soluble benzodiazepine that produces minimal irritation after IV or intramuscular (IM) injection. When exposed to physiologic pH, an intramolecular rearrangement occurs that changes the physiochemical properties of midazolam such that it becomes more lipid soluble.
2. Benzodiazepines undergo hepatic metabolism via oxidation and glucuronide conjugation. Oxidation reactions are susceptible to hepatic dysfunction and coadministration of other drugs such as H2-receptor antagonists.
a. The hepatic clearance rate of midazolam is five times greater than that of lorazepam and 10 times greater than that of diazepam.
b. Diazepam is metabolized to active metabolites, which may prolong its residual sedative effects.
c. Lorazepam is directly conjugated to glucuronic acid to form pharmacologically inactive metabolites. The primary metabolite of midazolam (1-hydroxy-methylmidazolam) has some CNS depressant activity.
d. The context-sensitive half-times for diazepam and lorazepam are very long, so only midazolam should be used for continuous infusion.
3. Benzodiazepines decrease CMRO2 and CBF analogous to the barbiturates and propofol, but these drugs have not been shown to possess neuroprotective activity in humans.
a. In contrast to other compounds, midazolam is unable to produce an isoelectric EEG.
b. Similar to the other sedative–hypnotic drugs, the benzodiazepines are potent anticonvulsants that are commonly used to treat status epilepticus.
4. Benzodiazepines produce dose-dependent depression of ventilation that is enhanced in patients with chronic respiratory disease, and synergistic depressant effects occur when benzodiazepines are coadministered with opioids.
5. Both midazolam and diazepam produce decreases in systemic vascular resistance and systemic blood pressure (accentuated with hypovolemia) when large doses are administered for induction of anesthesia, but a ceiling effect appears to exist above which little further change in arterial pressure occurs.
6. Short-acting IV sedatives are characterized by water-soluble benzodiazepines with full agonist activity and a higher plasma clearance rate compared with midazolam.
7. In contrast to all other sedative–hypnotic drugs, there is a specific antagonist for benzodiazepines. (Flumazenil has a high affinity for CNS benzodiazepine receptors but possesses minimal intrinsic activity.)
a. Flumazenil acts as a competitive antagonist in the presence of benzodiazepine agonist compounds.
b. Recurrence of the CNS effects of benzodiazepines may occur after a single dose of flumazenil (which is rapidly metabolized in the liver with an elimination half-time of about 1 hour) because of residual effects of the more slowly eliminated agonist.
c. In general, 45 to 90 minutes of antagonism can be expected after IV administration of 1 to 3 mg of flumazenil. (Sustained effects require repeated doses or continuous infusion.)
D. Etomidate
1. Etomidate is a carboxylated imidazole (only the dextro isomer possesses anesthetic activity) that is structurally unrelated to any other IV anesthetic, but similar to midazolam (which also contains an imidazole nucleus), etomidate undergoes an intramolecular rearrangement at physiologic pH, resulting in a closed ring structure with enhanced lipid solubility.
a. This drug is formulated with propylene glycol, contributing to a high incidence of pain on injection and occasional venoirritation.
b. The standard induction dose of etomidate (0.2–0.4 mg/kg IV) produces a rapid onset of anesthesia (myoclonic movements are common because of an alteration in the balance of inhibitory and excitatory influence on the thalamocortical tract), and emergence is prompt. (Extensive ester hydrolysis in the liver, forming inactive water-soluble metabolites.)
c. The frequency of myoclonic-like activity can be attenuated by prior administration of opioid analgesics, benzodiazepines, or remifentanil or administration of small sedative doses of etomidate (0.03–0.05 mg/kg) before induction of anesthesia.
2. Analogous to the barbiturates, etomidate decreases CMRO2, CBF, and ICP, but the hemodynamic stability associated with etomidate maintains adequate cerebral perfusion pressure.
a. An inhibitory effect on adrenocortical synthetic function (a single dose inhibits 11-β-hydroxylase for 5 to 8 hours) limits its clinical usefulness for long-term treatment of increased ICP.
b. Although an anticonvulsant effective in terminating status epilepticus, etomidate is also capable of evoking EEG evidence of seizure activity in attempts to identify seizure foci.
c. Etomidate produces a significant increase in the amplitude of somatosensory evoked potentials and can be used to facilitate the interpretation of somatosensory evoked potentials when the signal quality is poor.
3. Etomidate causes minimal depression of ventilation and cardiovascular function (it may be recommended for induction of anesthesia in poor-risk patients with cardiopulmonary disease) and does not release histamine (it is an acceptable drug choice for use in patients with reactive airway disease).
4. Etomidate is associated with a high incidence of nausea and vomiting, especially if it is combined with an opioid.
5. Etomidate may inhibit platelet function and prolong bleeding time.
E. Ketamine
1. Ketamine is an arylcyclohexylamine that is structurally related to phencyclidine.
a. The commercially available preparation is a racemic mixture, although the S+ isomer possesses more potent anesthetic and analgesic properties, reflecting its fourfold greater affinity at the binding sites on the NMDA receptor. Hepatic biotransformation of the S+ isomer is more rapid, contributing to a faster return of cognitive function. Both isomers possess similar cardiovascular-stimulating properties, and the incidence of dreaming is similar with the S+ isomer and the racemic mixture.
b. Ketamine produces dose-dependent CNS depression, leading to a so-called “dissociative anesthetic state” characterized by profound analgesia and amnesia. Low-dose ketamine (75–200 μg/kg/min IV) produces opioid-sparing effects when administered as an adjuvant during general anesthesia.
c. Induction of anesthesia can be accomplished with 1 to 2 mg/kg IV (4–8 mg/kg IM), producing an effect that lasts for 10 to 20 minutes, although recovery to full orientation may require an additional 60 to 90 minutes.
d. Subanesthetic doses of ketamine (0.1–0.5 mg/kg IV) produce analgesic effects. A low-dose infusion of ketamine (4 μg/kg/min IV) is equivalent to morphine (2 mg/hr IV) for production of postoperative analgesia.
2. Ketamine is extensively metabolized in the liver to norketamine, which is one third to one fifth as potent as the parent compound.
3. Psychomimetic reactions during the recovery period from ketamine anesthesia are less likely in patients also treated with benzodiazepines, barbiturates, or propofol.
4. Ketamine increases CMRO2, CBF, and ICP (which is minimized by hyperventilation of the lungs and pretreatment with benzodiazepines).
5. Ketamine can activate epileptogenic foci in patients with known seizure disorders but otherwise appears to possess anticonvulsant activity.
6. Ketamine is often recommended for induction of anesthesia in patients with asthma because of its ability to produce bronchodilation.
a. Depression of ventilation is minimal in clinically relevant doses.
b. Increased oral secretions may contribute to the development of laryngospasm.
7. Ketamine has prominent cardiovascular-stimulating effects (increased blood pressure, heart rate, pulmonary artery pressure) most likely because of direct stimulation of the sympathetic nervous system. This is possibly undesirable in patients with coronary artery disease.
a. Ketamine has intrinsic myocardial depressant properties, which may only become apparent in seriously ill patients with depleted catecholamines.
b. Ketamine can reduce the magnitude of redistribution hypothermia owing to its peripheral arteriolar vasoconstriction.
8. The renewed interest in ketamine is related to the use of smaller doses (100–250 μg/kg) as an adjuvant during anesthesia.
a. The anesthetic (sedative) and opioid analgesic-sparing effects of ketamine may reduce ventilatory depression during monitored anesthesia care.
b. The availability of the stereoisomer of ketamine has increased the nonanesthetic adjunctive use of ketamine. The anesthetic and analgesic potency of S(+) ketamine is three times greater than R(–) ketamine and twice that of the racemic mixture.
F. Dexmedetomidine
1. Dexmedetomidine is a highly selective α2-agonist that produces sedation and analgesia with less depression of ventilation than other commonly used drugs. The drug is approved for sedation of mechanically ventilated patients in the intensive care unit (ICU) but is also being used during diagnostic and therapeutic procedures (regional and local anesthesia) outside the ICU (“off-label use”).
2. When used for premedication, dexmedetomidine is comparable to midazolam, but the incidence of intraoperative hypotension and bradycardia is increased.
3. The acute hemodynamic response to laryngoscopy and intubation is blunted by dexmedetomidine. Dexmedetomidine may improved intraoperative hemodynamic stability and facilitate postoperative pain management.
III. CLINICAL USES OF INTRAVENOUS ANESTHETICS
A. Use of Intravenous Anesthetics as Induction Agents (Table 18-5)
1. Propofol has become the IV drug of choice for outpatients undergoing ambulatory surgery.
2. Clinical use of midazolam (often combined with other injected drugs), etomidate (cardiac stability), and ketamine (hypovolemic patients) is restricted to specific situations in which their unique pharmacologic profiles offer advantages over other available IV anesthetics.
TABLE 18-5 INDUCTION CHARACTERISTICS AND DOSAGE REQUIREMENTS FOR INTRAVENOUS ANESTHETIC DRUGS
0 = no change; + = increase; – = decrease.
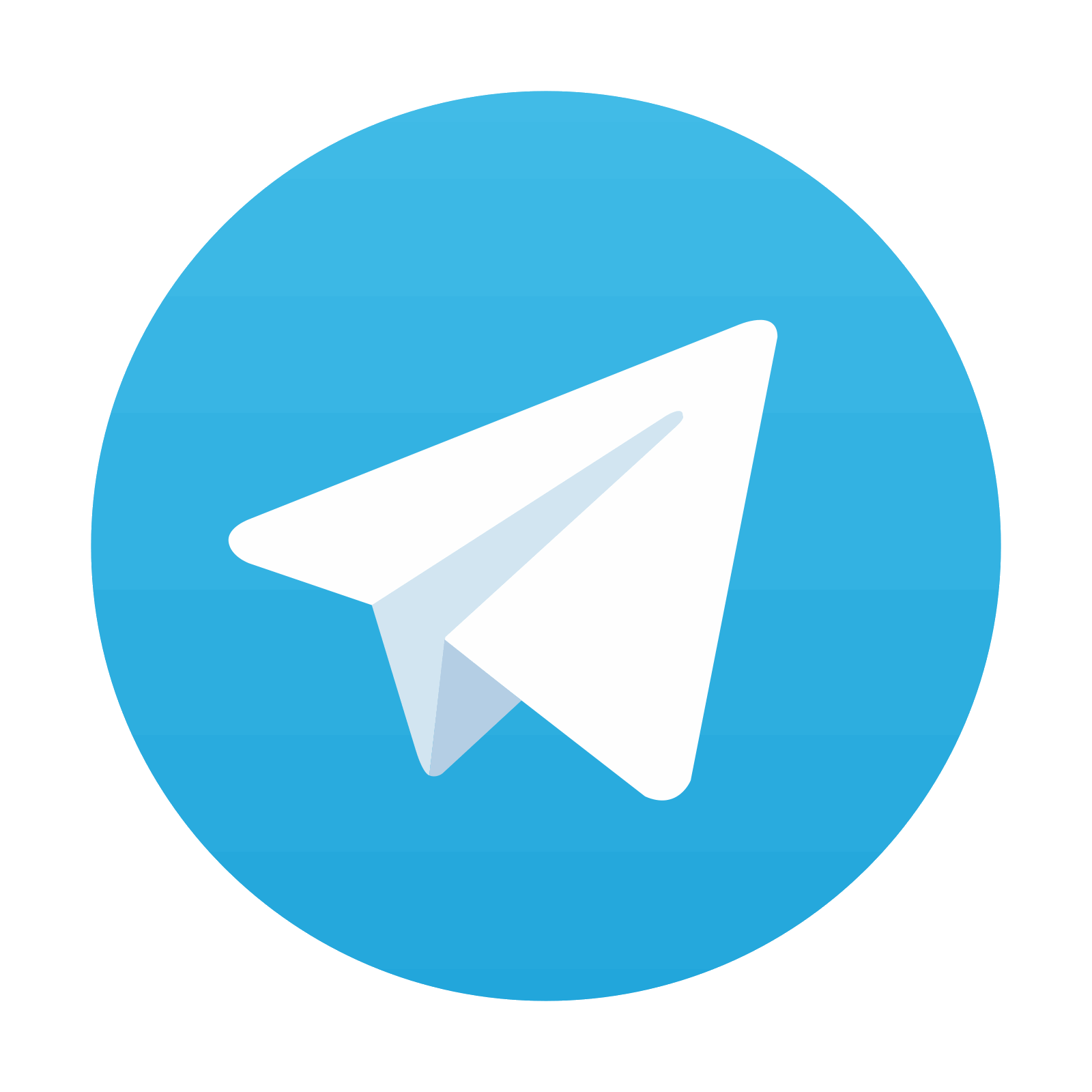
Stay updated, free articles. Join our Telegram channel
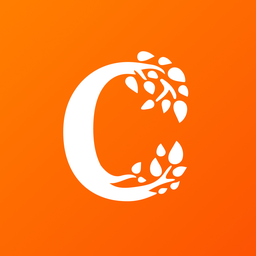
Full access? Get Clinical Tree
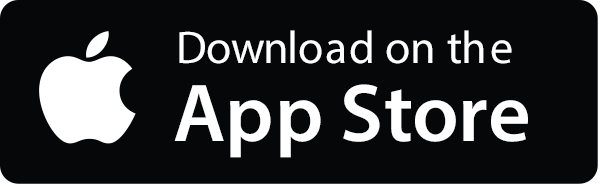
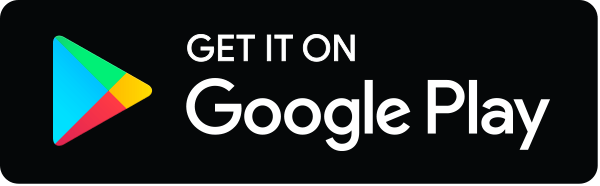