Pearls
- •
As intracranial hypertension progresses, changes may occur in the vital signs, with an elevation of blood pressure, decrease or increase in pulse, and irregularity in the respiratory rhythm. These signs, sometimes associated with episodes of decerebrate rigidity, indicate the occurrence of transtentorial herniation—or “coning”—that will lead to death if the process cannot be reversed.
- •
The continuous measurement of intracranial pressure is an essential modality in most brain monitoring systems. After a decade of enthusiastic attempts to introduce newer modalities for brain monitoring (e.g., tissue oxygenation, microdialysis, cortical blood flow, transcranial Doppler ultrasonography, and jugular bulb oxygen saturation), the measurement of intracranial pressure remains a robust and only moderately invasive modality that can be realistically conducted in most pediatric critical care units.
In most organs in the human body, the environmental pressure for blood perfusion is either low or coupled to atmospheric pressure. The environmental pressure for the brain differs in this respect because the brain is surrounded and protected by a stiff skull. Thus, a rise in environmental pressure—intracranial pressure (ICP)—may impede cerebral blood flow (CBF) and cause ischemia. In pediatric critical care, ICP may be of acute significance in a number of instances (e.g., traumatic brain injury [TBI], bacterial meningitis, hydrocephalus, and the Fontan circulation). In this chapter, we discuss how information from ICP monitoring helps our understanding and treatment of brain disorders.
Clinical background
In critical illness, the early recognition and treatment of intracranial hypertension is important because it is a major cause of mortality and morbidity. Therefore, an attempt should be made to collate the clinical evidence for and against its presence. However, the early symptoms and signs of this complication, which are invariably subtle and nonspecific ( Table 63.1 ), make this form of assessment somewhat limited. As will be discussed later, as intracranial hypertension progresses, changes may occur in the vital signs, with an elevation of blood pressure, decrease or increase in pulse, and irregularity in respiratory rhythm. These signs, sometimes associated with episodes of decerebrate rigidity, indicate the occurrence of transtentorial herniation (or “coning”) and imply the possibility of impending death if the process cannot be reversed. Unfortunately, recognition at this stage is often too late to prevent death.
Infant | Child | |
---|---|---|
General state |
|
|
Head/eyes |
|
|
Other |
|
|
Brain tissue shifts may produce various syndromes (see Chapter 62 ). First, transtentorial or cerebellar herniation may result in midbrain or medullary compression. Many of the clinical signs observed in association with herniation result from direct compression of structures by the impacted tissue or are due to angulation of nerves or arteries against normal structures in the area. These herniations can cause increasing coma, with distortion of the brainstem leading to midbrain and pontine hemorrhages. Cerebellar herniation is likely to occur when the increase in ICP is maximal in the posterior fossa. Such herniation occurs more commonly downward, squeezing one or more of the cerebellar tonsils through the foramen magnum; compressing the medulla; and leading to neck stiffness, head tilt, lower cranial nerve palsies, respiratory irregularities, or sudden cardiorespiratory arrest. Cerebellar herniation may occur upward through the tentorial notch, causing midbrain compression and leading to paralysis of upward gaze, dilated and fixed pupils, and respiratory abnormalities, although this type of cerebellar herniation is uncommon.
When intracranial hypertension is more marked in the supratentorial compartment—for instance, with acute intracerebral hematoma—the temporal lobe on the affected side may be displaced into the tentorial notch and result in unilateral transtentorial herniation. The herniation may be more marked anteriorly (uncal) or posteriorly (hippocampal) and is usually accompanied by displacement of the ipsilateral cingulate gyrus under the falx (cingulate herniation). Clinical manifestations of this condition may include ipsilateral third cranial nerve palsy, contralateral hemiparesis, respiratory irregularities, deepening coma with decerebrate posturing, and, ultimately, cardiorespiratory arrest.
Finally, when bilateral or a general increase in ICP in the supratentorial compartment occurs, as in diffuse cerebral edema, central transtentorial herniation may occur. This condition leads to impairment of upward gaze, pupillary constriction, hypertonus, and decerebrate posturing. Temperature irregularities and diabetes insipidus may develop; eventually, cardiorespiratory arrest may occur. A summary of the clinical features of “central syndrome” is provided in Table 63.2 .
Stage | Level of Consciousness | Respiration | Pupil Size and Reactivity | Oculocephalic and Oculovestibular Responses | Posture and Tone |
---|---|---|---|---|---|
|
|
| Small (1–3 mm) with brisk reaction to light | Conjugate at rest and responds quickly |
|
| Coma | Central hyperventilation | Midposition (3–5 mm) with sluggish reaction to light | Dysconjugate | Decorticate posturing and increased tone |
| Deep coma | Midposition and fixed | Absent | Flaccid: (1) retained bilateral extensor plantars and (2) occasional flexor responses in the lower limbs | |
Medullary (terminal) | Deep coma | Irregular breathing interrupted by deep sighs, gasps, and then terminal apnea | May be unequal | Absent | Flaccid |
Physiology of the intracranial vault
A physiologic process underlies the clinical picture of intracranial hypertension. In this section of the chapter, our intention is to familiarize the pediatric intensivist with new approaches to understanding the hydrodynamic function of the intracranial vault. In other words, what happens before brain tissue shifts occur? In common with any aspect of physiology, the tasks have been straightforward: Can it be measured? Can it be modeled? How do the models help in the understanding of the underlying homeostasis and the mechanism of derangement and perturbation? We highlight these topics and hope that the reader will see the obvious application to pediatric critical care—that is, use of this form of cerebral monitoring and potential approaches to ICP assessment. In many specific conditions, however, knowledge is still lacking with regard to which parts of the adult-generated theory may be fully applicable to children.
Intracranial pressure
The brain is an expansile structure that expands and contracts with each beat of the heart. Because there are no valves within the venous drainage from the brain, any changes in intrathoracic pressure are transmitted to ICP. Such phenomena can be seen and palpated simply through the examination of an infant’s fontanelle and quantified with cerebrospinal fluid (CSF) pressure recording. Once the cranial sutures have fused, any change in cerebral blood volume (CBV) on the arterial-to-arteriolar side of the cerebral circulation must be compensated by either reduction in cerebral venous volume or by phasic movement of CSF out of the intracranial vault through the foramen magnum. Such expansion of the cerebral mantle with compression of the lateral ventricles during systole and movement of CSF through the aqueduct of Sylvius and to-and-fro through the foramen magnum was first visualized with pneumoencephalography. These changes now can be seen more easily with dynamic magnetic resonance imaging (MRI). Inevitably, a lag phase exists between the systolic increase in CBV and the effect of the compensatory mechanisms so that CSF pressure increases to reflect, in part, the systolic waveform. The CSF pressure waveform is not an exact replica of the arterial waveform because it has been “filtered” by the combined effects of arterial wall compliance of the cerebral arteries, cerebrovascular resistance, and intracranial compliance ( Fig. 63.1 ).

Utility of hydrodynamic and electrical analog models of intracranial pressure
ICP is a function of the circulation of CBF and CSF in which ICP is related to a vascular component (ICP vascular ) and a CSF component (ICP CSF ). There is considerable interest in modeling these relationships as an aid to understanding some of the complex phenomena seen in critically ill patients. The vascular component is difficult to express quantitatively. However, multiple variables—such as arterial blood pressure (ABP), autoregulation, and cerebral venous outflow—all contribute to it. Regarding the other component, circulation of CSF, up to 80% of CSF is the product of active secretion by the choroid plexus, and movement of interstitial fluid into the ventricles and subarachnoid space contributes the remainder in the uninjured brain. Drainage is largely passive via arachnoid villi and granulations into the superior sagittal sinus and spinal root sleeve venous drainage. Some drainage, which is currently unquantifiable, occurs through the olfactory bulb and mucosa into the deep cervical lymphatics.
The equation by Davson and colleagues shows the immediate relationships controlling CSF pressure:
With these two components—vascular and CSF—taken together, Fig. 63.2 shows the electrical analog circuit equivalent of CBF and CSF circulation. Such models can be used to interrogate ICP and CSF dynamics clinically. These models can also be used to estimate ICP noninvasively. For example, Kashif et al. described a model-based noninvasive estimation of ICP from middle cerebral artery CBF velocity using transcranial Doppler (TCD) and arterial pressure from 35 hours of data in 37 adult patients with severe TBI. The authors generated ICP estimates and achieved a mean error (bias) of 1.6 mm Hg. More recently, Fanelli et al. evaluated a similar model in 12 pediatric and young adult patients (aged 2–25 years) and described a method for real-time estimation of noninvasive ICP with values falling within ±7 mm Hg of the reference ICP measurement ( Fig. 63.3 ). Overall performance gave a mean error (bias) of 1.0 mm Hg, standard deviation of the error (precision) of 5.1 mm Hg, and root-mean-square error of 5.2 mm Hg. The associated mean and median absolute errors were 4.2 mm Hg and 3.3 mm Hg, respectively.


The assessment of ICP is needed to direct medical interventions in infants, children, and adolescents with severe TBI. , However, there are instances in which physicians do not carry out invasive ICP monitoring but being able to follow it in real-time would be useful, for example, in moderate-severity TBI, certain cases of craniosynostosis, metabolic disorders, central nervous system (CNS) infection, fulminant liver failure, and certain other forms of acute encephalopathy. , It has been suggested that noninvasive ultrasound measurement of the optic nerve sheath diameter (ONSD) may be used to identify and monitor elevated ICP. Unfortunately, such measurements are neither suitable for continuous real-time monitoring nor are they able to provide absolute ICP. However, one approach used in adults in the emergency department is to measure ONSD in order to give some indication of whether ICP is elevated or not. Patient-specific ICP determination would be a key advancement for the care of children, particularly in the range of values when symptoms are not obvious: 15 to 25 mm Hg. Of note, the technique described by Fanelli et al. performs robustly in the ICP range 15 to 25 mm Hg, which—if supported by advancements in noninvasive ABP technology and miniaturization of TCD technology —could be transformative to practice. Just consider surveillance and interventions that would be possible beyond the care of pediatric patients with severe TBI to a number of coma-inducing conditions complicated by intracranial hypertension worldwide, such as CNS infection.
Cerebral vasodilation and cerebral spinal fluid pressure
Three major factors regulate CBF: cerebral perfusion pressure (CPP), partial pressure of arterial carbon dioxide (Pa co 2 ), and partial pressure of arterial oxygen (Pa o 2 ). Hypercapnia causes cerebral vasodilation, increases CBF, and increases ICP. Hypoxia also causes cerebral vasodilation and a rise in ICP. CPP is taken as the difference between mean ABP and ICP and represents the pressure gradient acting across the cerebrovascular bed. Therefore, it is an important factor in regulation of CBF. Under normal circumstances, over a wide range of CPP, CBF is autoregulated (i.e., it remains constant when CPP varies). Thus, regarding the effect of this phenomenon on ICP, active cerebral arteriolar constriction occurs and ICP consequently falls to maintain CBF when ABP is increased (i.e., hypertension). At the other extreme, systemic hypotension (within the autoregulatory range) provokes cerebral vasodilation and an increase in ICP. When autoregulation is defective, ICP increases and decreases with AB—that is, it is pressure passive.
In practice, measurement of ICP can be used to estimate the CPP when it is the most significant downstream pressure acting on vascular perfusion. However, in some instances, venous pressure may be of more significance, such as critical Fontan circulation.
Cerebral perfusion pressure and cerebral autoregulation
In adults, the lower limit for CPP is taken as a threshold of 60 to 70 mm Hg. In children, however, it is evident from measurement of normal ABP that the lower limit for CPP must be lower than this adult level for much of childhood ( Fig. 63.4 ).

To date, two general approaches are used to study indirectly whole brain circulation pathophysiology. First, there is an empiric approach using observational and epidemiologic evidence to establish statistical relationships and correlations between inputs and outputs. This approach is best exemplified by studies in severe TBI using PR x (i.e., pressure reactivity index in which 5–7 minutes of time-averaged ICP and ABP data are used to calculate mean Fisher-transformed Pearson correlation) to derive, by statistical analyses, the CPP optimal for an individual (i.e., CPP Opt ). Briefly, the assumption in this approach is that when vascular reactivity is absent, ABP and ICP are positively correlated. By comparison, strong cerebrovascular vasomotor constriction in response to increased ABP results in decreased CBV, which leads to decreased ICP and negative PR x ( Fig. 63.5 ). The CPP Opt is defined as the CPP at which PR x is at its minimum ( Fig. 63.6 ). In severe TBI, the difference between contemporaneous time-series median CPP (CPP Med ) and CPP Opt (i.e., Δ CPP ) 0 to 5 mm Hg is associated with favorable outcome, whereas Δ CPP –10 to –15 mm Hg occurs only in those with unfavorable outcome. Such empiric associations and observations do not provide better understanding of pathophysiology or even the determinants of CPP Opt . PR x -derived CPP Opt , with calculation of Δ CPP and Δ Category , are basically statistical constructs that have proven value in patient risk-stratification and in epidemiologic association with poor outcome in adult cases of severe TBI. , The main reason here is that CPP is not a physiologically controlled parameter in homeostasis; there is no sensor, no error signal, no set point or optimum within the operating range between the upper and lower limits of cerebral autoregulation. Hence, CPP Opt -guided management may be a testable pragmatic therapeutic approach for future clinical trials but, intrinsically, empiricism has limitations to understanding mechanistic functions.
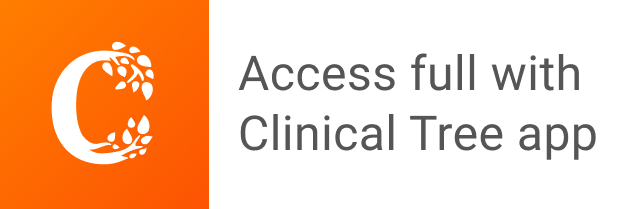